the Creative Commons Attribution 4.0 License.
the Creative Commons Attribution 4.0 License.
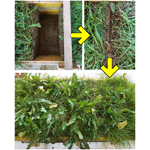
Technical Note: Combining undisturbed soil monoliths for hydrological indoor experiments
Peter Strauss
An important decision in soil hydrological research is whether to conduct experiments outdoors or indoors. Both approaches have their advantages and trade-offs. Using undisturbed soil monoliths combines some of the advantages of outdoor and indoor experiments; however, there are often size limitations. Acquiring large monoliths necessitates heavy machinery, which is time-, cost-, and labor-intensive. Small- to medium-sized soil blocks, however, can be obtained using less demanding methods. A promising approach is the combination of smaller blocks to form a single large monolith, thereby optimizing cost and labor efficiency as well as representativity and upscaling potential. To this end, we compared the runoff properties of medium-sized ( m) grassland soil monoliths cut in half and recombined with uncut blocks. We conducted artificial runoff experiments and analyzed the chemical composition and amount of outflow from four flow pathways (surface runoff, subsurface interflow, percolating water, lateral flow). Furthermore, we studied surface runoff velocity parameters using a salt tracer. Our results suggest that the effects of the recombination procedure are negligible compared to the variation in the data caused by the inherent soil heterogeneity. We propose that the benefits of combining soil monoliths outweigh the potential disadvantages.
- Article
(4500 KB) - Full-text XML
-
Supplement
(4324 KB) - BibTeX
- EndNote
An important question in soil hydrological research is whether to conduct experiments outdoors or indoors, i.e., in situ or ex situ. Both are frequently used in artificial rainfall or runoff experiments (e.g., examining erosion or nutrient export) and have each specific strengths and weaknesses. The main advantage of outdoor experiments is that the studied soils have developed naturally and are fully integrated into the surrounding landscape. They are shaped by physicochemical processes and biological activity and, thus, have developed three-dimensional characteristics (e.g., vertical gradients, macropore network, root system) that cannot easily be reproduced artificially (Green, 2014; Katagi, 2013). Accordingly, the results obtained have an inherent real-life relevance. A downfall is that it can be challenging to find sites with desired conditions, especially ones that are homogeneous over a larger area, so replicate plots can be installed. Frequently, there is only a narrow time frame of constant weather conditions, especially concerning temperature and precipitation (Kuhn et al., 2014).
The main advantages of indoor trials are more control over variables and independence from the weather. Furthermore, there is the possibility of testing the same soil under different situations, which would be difficult or downright impossible in the field, for example different slopes. Another significant benefit is better access to infrastructure, resources, and measuring instruments, saving time and work required (Douglas et al., 1999). Indoor experiments may also be preferred in studies that examine nutrients or pollutants, as these can be collected and discarded without getting into the environment. On the other hand, it is challenging to simulate outdoor conditions with indoor experiments, especially if disturbed soil is used and vegetation is grown artificially (Johnson et al., 1995; Poorter et al., 2016). Owing to limited plot sizes, there is also the question of to what extent results can be extrapolated to relevant larger scales (Lewis and Sjöstrom, 2010).
Using undisturbed soil monoliths combines some of the advantages of outdoor and indoor experiments: naturally developed soils combined with high flexibility and control over variables. For upscaling purposes and a more accurate representation of soil processes and their variability, it would be desirable to use soil volumes that are as large as possible; however, the amount of work needed increases substantially with size. The collection of large monoliths (over 1 m3) necessitates heavy machinery such as hydraulic rams, excavators, and cranes (Belford, 1979; Darch et al., 2015; Schneider et al., 1988). For smaller monoliths it is possible to use methods that require only minimal use of technical gear. For instance, push methods can be applied in which a sampling frame is driven into the ground with a mallet, or a frame may be built around a pre-cut soil volume (Douglas et al., 1999; Palmer et al., 2011). Furthermore, the less heavy the monoliths are, the easier they are to handle, store, and discard (Allaire and van Bochove, 2006). Cylindrical soil monoliths are often used for lysimeters, while runoff and erosion studies commonly employ rectangular blocks (Allaire and van Bochove, 2006; Douglas et al., 1999). A promising approach for runoff research appears to be taking two or more equally sized smaller monoliths at a site and combining them into a single large block, thereby optimizing cost and labor efficiency as well as representativity and upscaling potential. However, the contact areas between the individual monoliths may affect runoff and transport processes, such as infiltration and sediment movement.
Here, we report on the potential to combine undisturbed soil monoliths to acquire larger soil units for studying runoff and nutrient transport. To this end, we collected six monoliths ( m) in grassland, representing vegetated filter strips (Prosser et al., 2020). Three monoliths were cut in half and recombined again, and the others remained uncut. We conducted artificial runoff experiments with tracer applications and flow velocity measurements to examine whether recombined and uncut blocks behave differently. In principle, the contact zone between two individual blocks could act as a large macropore, promoting preferential flow and, thus, a higher share of percolating water at the expense of surface runoff. However, our main hypothesis was that – done properly – the recombination procedure has no directional effect on runoff properties. Accordingly, we hypothesized that (1) recombined monoliths do not differ regarding the amount of outflow at the different flow pathways or their proportional share, (2) recombined monoliths do not show a faster onset of percolating water or (3) a faster increase in tracer concentration within the percolating water, and (4) there is no difference in surface runoff velocity between treatments. Additionally, we discuss general issues related to indoor runoff experiments.
2.1 Monolith sampling and preparation
The six monoliths were taken from a permanent grassland near Wieselburg, Lower Austria, Austria (see Table 1 for main soil properties). We used a push method for monolith collection, similar to the method used by Tiefenbacher et al. (2021). A custom-built steel frame ( m) with a cutting edge was placed on the soil surface and driven into the ground using body weight and a mallet. To ease penetration and minimize compaction and disturbance, the soil around the frame was gradually removed with spades. Once the desired depth was reached, a bottom plate was inserted with a rack and pinion jack. The frame was towed onto a trailer using wooden ramps and an electrical winch. In the workshop, the monoliths were transferred to plywood boxes. Three of the monoliths were cut in half vertically to obtain two m sized blocks. The blocks were interchanged so that the left front of the left block faced the right front of the right block. They were then recombined by applying a viscous soil–water mixture to the facing fronts and tightening the fit of the plywood box (see Sect. S1 in the Supplement for details on sampling and cutting). For a proper recombination, the blocks must be of equal width and depth. Furthermore, the monoliths, and the soil used for the soil–water mixture, need to be taken from the same site close to one another. Monoliths were watered regularly.
2.2 Experimental setup
The runoff experiments were carried out in two experimental sets, each comprising a full round of experiments (runoff and tracer measurements) on all six monoliths. During the first set, two flow pathways were recorded at the lower end of the monolith: the surface runoff and subsurface interflow. For the second set, we further sampled and distinguished between percolating water that went through the whole soil body vertically and water that infiltrated the soil body, but left the monolith again at the side due to lateral flow pathways.
The setup consisted of an overflow tank, a steel frame that allowed the collection of surface water, and a horizontal plate inserted at the middle of the block to collect subsurface interflow. For the second set a bottom steel frame was also used for the collection of percolating water and lateral flow (Fig. 1). The frames were 2 cm smaller than the monoliths on each side, preventing both runoff water from being drained by a gap between the box and the monolith and lateral flow from getting into the collector for percolating water. As a precautionary measure, we sealed the potential space between the frame and the soil with a sodium silicate solution (“water glass”; see Sect. S2 for preliminary experiments on applicability). The inclination was based on typical slopes of vegetated filter strips (VFSs) in Austria and was adjusted to 3 % during the first set and 4 % during the second set.
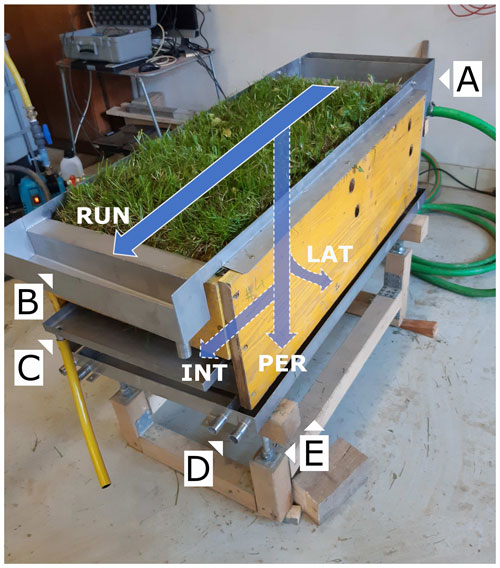
Figure 1Setup of runoff experiments with flow pathways (arrows). [A] Overflow tank; [B] metal frame with surface runoff (RUN) collector; [C] metal plate for subsurface interflow (INT) collection; [D] bottom frame with collectors for percolating water (PER; inner outlets) and lateral flow (LAT; outer outlets); [E] rack with slope-adjustable gear.
Before each experiment, the monoliths were transferred to a water pool until fully saturated and then left to drain for 24 h to obtain comparable field capacity conditions for the soil water content (see Tiefenbacher et al., 2021). Each experimental set comprised three phases. During the first phase, an electric pump distributed runoff over the monolith through the overflow tank. A constant flow of 5 L min−1 was applied using a valve and water meter. Deionized water spiked with orthophosphate (0.5 mg L−1) was applied to mimic typical local agricultural runoff, as was bromide (∼700 mg L−1) as a conservative tracer. Outflow was collected and measured using buckets, which were exchanged every minute during the first 10 min after the onset of an outflow and afterwards in increasing intervals. Additional samples were taken for chemical analysis after approximately 2, 5, 10, and 30 min. The subsurface interflow was very low; as the chemical analyses required a minimum amount of water, taking samples often took substantially longer than the 1 min interval, and not all samples could have been taken. The second phase started after 45 min and lasted for approximately 15 min, during which surface flow velocity (cm s−1) measurements were carried out in three replicates for each monolith. For this, 10 mL of a potassium chloride solution (7.455 g KCl L−1; 12 900 µS) was applied at the upper end of the monolith, and the conductivity at the overflow tank (baseline value) and in the surface runoff collector was monitored (see Sect. S3 for details). In the last phase, the monoliths were flushed with deionized water for 60 min to remove physically retained chemicals.
2.3 Chemical and statistical analysis
Water samples were analyzed for bromide and phosphate concentration. Bromide was determined by ion chromatography, and soluble orthophosphate was determined photometrically, following national standards.
Nonparametric Kruskal–Wallis tests on the sum of outflow values were conducted to check for statistically significant differences between treatments. Bonferroni-adjusted Dunn's post hoc tests were used to localize significant differences between individual monoliths. To account for slightly different inflow rates between monoliths, a standardized outflow value was calculated by dividing the measured outflow rate at a flow pathway by the actual inflow rate. For the statistics and box plots, discharge values for each flow pathway for every minute were calculated from the raw data, and only values between minutes 5 and 45 were used to eliminate the initial phase wherein flow rates were not yet stable. For the tracer experiments, the velocity of the leading edge and the centroid was calculated following Abrantes et al. (2018). Figure generation and statistical testing were carried out using Python 3.9.12 embedded in the Spyder 5.1.5 environment. Libraries used were scipy, scikit_posthoc (statistics), matplotlib, seaborn (figures), numpy, and pandas (data handling). Statistical significance was set at the α=0.05 level.
Here, we only report results from the second experimental set. Details on the first set can be found in the Supplement (Sect. S4) but are referred to when deemed appropriate.
Table 2Results of outflow measurements. Time to runoff gives the time until runoff was recorded for the respective flow pathway. Share of total water exports gives the percentage that each flow pathway contributes to the total outflow from a monolith. Total water budget is the difference between the applied runoff and the sum of all outflow for each monolith (i.e., a positive value means a net water uptake, negative values a net water release).
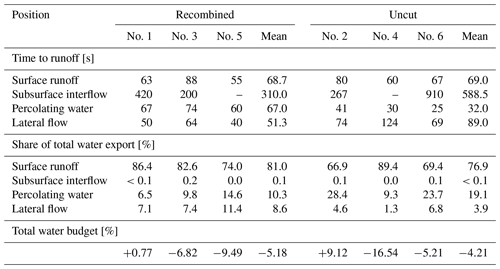
3.1 Water flow
Generally, surface runoff, percolating water, and lateral flow responded quickly to the runoff application; the time until outflow was recorded was commonly around 1 min. Both treatments had a similar beginning of surface runoff outflow at around 69 s, but recombined blocks had a 38 s earlier onset of lateral flow and 35 s later onset of percolating water on average. Subsurface interflow was always the latest to start (Table 2).
Irrespective of treatment, surface runoff always contributed the most to total water outflow at each monolith, followed by percolating water and lateral flow. Generally, subsurface interflow was very low; the highest share was 0.4 %, while two blocks had no subsurface outflow. For recombined monoliths, lateral flow contributed more to total outflow compared to uncut blocks (Table 2).
A similar overall picture was found for standardized outflow, with surface runoff having the highest outflow, followed by percolating water, lateral flow, and subsurface interflow (Fig. 2). No significant differences between recombined and uncut blocks were found for surface runoff (H=0.43, P=0.51) and percolating water (H=2.33, P=0.13). Lateral flow was slightly below statistical significance (H=3.86, P=0.049) and tended to have a higher outflow (Fig. 2). However, there is an overlap of recombined and uncut blocks, and post hoc tests revealed high heterogeneity in the data: significant differences between blocks of the same treatment, and, vice versa, insignificant differences between blocks of different treatments were found for lateral flow and all other flow pathways (Sect. S5). Statistical testing was not feasible for subsurface interflow due to monoliths with zero outflow. Uncut blocks exhibited substantially higher within-group variances for surface runoff and lateral flow.
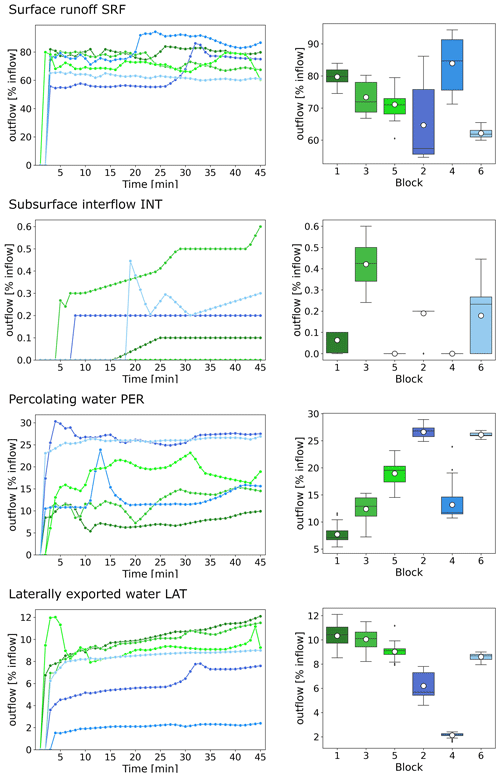
Figure 2Outflow rate at the respective flow paths. Green – recombined blocks, blue – uncut blocks. Different shades denote different blocks. Note that box plots integrate over minutes 5 to 45. White circles – mean; black line – median; box – 25–75th percentiles; whiskers – 5–95th percentiles; diamonds – outliers.
Four of the six monoliths had a higher water uptake than outflow, but no trend between recombined and uncut blocks was discernible, again due to substantial inner-group variation (Table 2).
3.2 Bromide and phosphate
Bromide concentration in the outflow increased with time, approaching 100 % of inflow concentrations. Some blocks also showed bromide concentrations slightly higher than 100 % (Sect. S6). Bromide concentrations were high right from the first measurements (i.e., 2 min after the onset of outflow), irrespective of the flow pathway. The lowest initial bromide concentration was 77 % of inflow bromide concentration (block no. 2, lateral flow); the highest initial concentrations were 98 % (block no. 5, lateral flow) and 99 % (block no. 1, surface runoff). There was a tendency for phosphate concentrations in the outflow to decrease with time when they were initially higher than the inflow phosphate concentration, as well as a tendency to increase when they were lower, in both cases approaching 100 % of the inflow phosphate concentration. Phosphate enrichment in the outflow was substantial, with up to more than twice the inflow concentration for particular samples (Sect. S6). No directional difference between recombined and uncut monoliths was found for bromide or phosphate concentration.
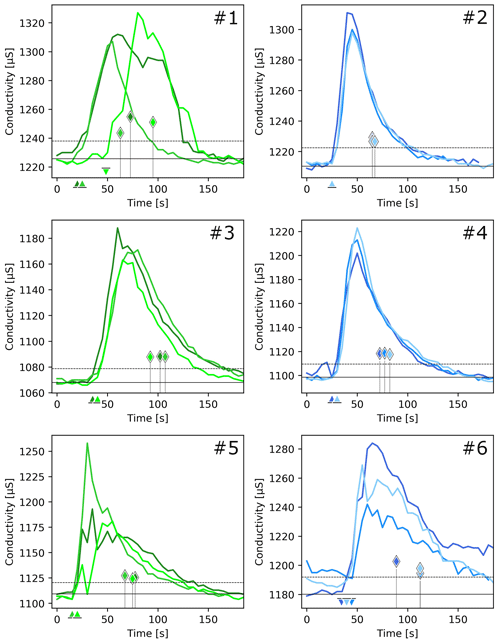
Figure 3Amplitude passage of salt tracer experiments. Green – recombined blocks, blue – uncut blocks. Different shades indicate different replicate trials (1–3). Triangles denote the time point of leading-edge passage; diamonds denote centroids. Black line – quiescent value; dashed line – threshold for the leading edge (see Sect. S3 for details).
3.3 Salt tracer
Although some blocks showed reasonably consistent results in the tracer experiment (e.g., block nos. 2–4), there was also substantial inner-block (replicates) and inner-group (treatment) variation (Fig. 3). Consequently, there is significant overlap and no directional difference identified between recombined and uncut monoliths regarding leading-edge or centroid velocity (Sect. S7).
4.1 General remarks
For a successful combination, it is vital that the single blocks are of equal width and height. While the sampling device usually fixes the width, acquiring similar heights may be more difficult. Uneven soil surfaces produce small barriers or ridges when monoliths are combined, which can interfere with runoff patterns. Furthermore, monoliths will have different heights at the front and back end if they are not taken at a right angle to the soil slope. Some adjustments can be made in the laboratory (e.g., different bottom plates to even height differences). Nevertheless, we strongly advise avoiding such issues in the first place by an a priori assessment of site conditions and careful sampling. This also includes a sampling design that allows the extraction of monoliths close to one another, limiting the effects of more significant scale gradients.
In principle, we expect the combination method to apply to a large variety of soil types, but we cannot yet provide textural limits. However, combining two soil blocks requires removing the bordering at one side. Thus, structurally weak soils that could collapse without a frame are not suitable for this method.
We recommend that soil monoliths be kept outside in a sheltered but sunny location. Blocks need to be watered regularly for plant vitality and to avoid drying and an emergence of cracks in the soil structure that would affect runoff properties and impede the repair of the combined monoliths (Bottinelli et al., 2016; Pires et al., 2007). As the blocks are isolated, they are much more prone to detrimental effects than they would be in situ. In practice, maintenance can be challenging: for instance, over weekends when the workshop or laboratory is vacant. As a general rule, a management plan becomes essential for experimental success if the monoliths are to be kept over a more extended period of time.
Another issue that concerns runoff experiments is the sealing of the soil body. Commonly, the gap between the soil monolith and the box or lysimeter wall is filled using resins, bentonite clay, foams, or other materials. The primary reason for this is to avoid water being drained via the gap and, thus, not interacting with the soil body (Singh et al., 2018). By filling the gap, the monolith is laterally sealed. In our study, we did not seal the gap between the box and the soil for two reasons: firstly, a direct drainage of water via the gap is already impeded by the frame, which is smaller than the monolith and forces the runoff to flow over – and into – the soil. Secondly, we aimed to mimic a vegetated filter strip (VFS). It is likely that the runoff enters a VFS in concentrated form due to flow convergence (Pankau et al., 2012; Ramler et al., 2022). In this scenario, only the VFS soil under the concentrated flow would receive runoff water, which could then infiltrate the soil and be laterally exported. In turn, this part of the soil would receive less water from the surrounding soil, which intercepts rain but no runoff water. Accordingly, we propose that this approach provides better conditions for our specific aims. We suggest that future runoff studies ponder whether sealing is appropriate or necessary.
4.2 Combining soil blocks
Generally, our main hypothesis that combining monoliths has no directional effect on the runoff properties was supported. Nevertheless, there was a trend of higher lateral outflow at recombined blocks, accompanied by a faster onset of lateral flow and a later onset of percolating water. It appears that the recombination procedure favors lateral flow and restricts percolating water outflow. We speculated that a potential difference between treatments would be caused by the contact area between two blocks functioning as an extensive macropore. This would promote preferential flow and quick drainage, leading to a higher amount of percolating water and lateral flow at the expense of surface runoff – which was not the case. One explanation for the higher share of lateral flow found for recombined monoliths would be macropores in the upper half of the contact area and a less permeable lower section, which would cause the macropore flow to be diverted sideways. Although this cannot be ruled out, we argue that it is not very probable that this happened.
Furthermore, there is no indication to reject the other hypotheses: the recombined blocks showed neither a faster rise of bromide concentrations nor a slower surface runoff velocity. We suspect that the observed differences were caused by generally high heterogeneity of the soils, low sample size (n=3), and stochastic effects, e.g., the quantity and orientation of macropores such as earthworm channels. For most variables, there was also substantial inter-group overlap and considerable within-group – and in some cases also within-block – variation. Moreover, the water budget of the monoliths and the results from the first experimental set (and partly from the water glass trials) provide similar results (Sects. S2 and S4). The direct comparison of both experimental sets further highlights the heterogeneity within the same monolith soil and the influence of repeated experimental procedures (Darch et al., 2015; Sharpley, 1997). However, this is not a peculiarity of our experiments but rather a common issue in soil research (Luk and Morgan, 1981). Sources of this natural variability of the soil are manifold, including vegetation patterns, edaphone activity, (micro-)relief, soil aggregation, and their often complex interactions with runoff (Boix-Fayos et al., 2006; Bryan and Luk, 1981). Furthermore, there may be anthropogenic impacts before, during, and after the monolith collection (Luk and Morgan, 1981; Rüttimann et al., 1995). The inherent variability can only be compensated for by increasing the sampling size to average the effects of microscale differences in the soil samples (Boix-Fayos et al., 2006; Bryan and Luk, 1981). Nevertheless, this also implies that the data noise added by the (re)combination procedure is negligible. We conclude that the recombination procedure did not lead to directional differences and, thus, had no adverse effect on runoff properties, suggesting that combining two (and probably more) blocks is a viable and practicable way to obtain single larger soil monoliths.
Irrespective of treatment, all flow pathways (except the subsurface interflow) had a rapid onset of outflow, commonly around 1 min after the start of the experiments, which can only be achieved through preferential macropore flow for the percolating water and lateral flow. This is further backed up by the high amounts of bromide and phosphate already in the first samples (taken after approx. 2 min), which shows that the emerging water originated primarily from the applied runoff and not from the water retained in the soil. As a side note, the enrichment of phosphate found for some blocks also demonstrates that VFS surface soils and subsoils can switch from phosphorous sinks to sources (Andersson et al., 2015; Reid et al., 2018). To examine the effect of macropores in more detail, we replaced the plywood walls of one box with transparent polycarbonate panes for an additional preliminary trial. It was apparent that most lateral outflow was restricted to specific outlets, i.e., through macropore channels that originate within the soil body and lead to the monolith edge (Video 1). Analogously, the same applies to percolating water.
It is possible that the soil texture was affected by transport and handling, though we argue that the macropores were already present in situ, e.g., through high activity of the soil fauna, as is characteristic for grassland soils (Lamandé et al., 2011; Menta, 2012). Active earthworms were continuously encountered throughout the experiment and were still found 9 months after sampling. Wormholes play an essential role in infiltration and can constitute a large share of the macropores within a soil, potentially generating a channel network with high flow rates (Roth and Joschko, 1991; Weiler and Naef, 2003). Furthermore, the collapse or sealing and, vice versa, the breakthrough and connection of earthworm channels (and other macropores; Jégou et al., 2002) may explain abrupt changes in outflow seen in some of the blocks (Fig. 2).
After the last experimental set, we left the monoliths without maintenance, which caused them to dry up completely. Thereby, the recombined monoliths cracked at the contact areas and developed a gap, exemplifying that complete consolidation (i.e., repair) did not happen. A proper merging of individual blocks into a single monolith is probably impossible to achieve, given the short duration of the experiment in relation to the bio-geochemical processes that govern soil development (Pires et al., 2007; Sarmah et al., 1996). Nevertheless, we argue that such a high level of “naturalness” is not necessary for runoff experiments; instead, it is sufficient that the combination procedure generates more advantages (e.g., better representativity of processes) than disadvantages (e.g., added data noise). Experiments, however, should not be conducted under dry soil conditions.
We used a comprehensive approach, analyzing runoff characteristics, chemical loadings, and flow velocity, and are, thus, confident in our conclusions. Nevertheless, there is room for further research, for instance applying different boundary and initial conditions, such as other soil types and flow rates, or a higher sample size and number of blocks to be combined. A comparison of large monoliths taken with heavy machinery with blocks of similar extent that are made up of combined smaller monoliths would be very interesting.
Working with undisturbed soil monoliths can be challenging and is always a compromise between available resources and sampling effort (e.g., sampling size, replicates, monolith dimensions). Combining medium-sized monoliths can help maximize the representativity and upscaling potential of experiments, while minimizing financial and labor efforts. There are, however, some aspects that have to be considered. Individual blocks need to be of equal width and height. Furthermore, proper storage and maintenance, especially regular watering, are crucial to keeping the monoliths in good condition and are, in turn, dependent on the research aim, the duration of the experiment, climate, and resources (e.g., staff, storage space). It is also important that the experimental setup matches the natural hydrological environment of the soil under investigation. In this study, for instance, we refrained from sealing the gap between the soil and box to mimic a grassland soil under concentrated flow (i.e., a vegetated filter strip).
We found general support for our initial hypotheses, as we have no indication that combining two soil monoliths has a directional effect on runoff properties. The observed differences between recombined and uncut blocks were against expectations and lacked a clear explanation. We conclude that the inherent heterogeneity of the soils – even if from the same site – is substantially more considerable and overshadow any effect of the combination procedure. Accordingly, the advantages outweigh the possible adverse effects, and we recommend combining monoliths for indoor runoff studies and related research. Nevertheless, we encourage further research on this subject to better delimit the potential and possible limitations of this procedure, for instance using different experimental setups (e.g., number of monoliths), boundary conditions (e.g., flow rates, soil types, dimensions), or analysis methods (e.g., X-ray imaging).
The original contributions presented in the study are included in the article and the Supplement; raw data and codes are available upon reasonable request from the corresponding author.
The supplement related to this article is available online at: https://doi.org/10.5194/hess-27-1745-2023-supplement.
DR and PS designed the study. DR conducted the experiments, analyzed the data, and took the lead in writing and overall organization of the paper. All authors contributed to paper revision and read and approved the submitted version.
The contact author has declared that neither of the authors has any competing interests.
Publisher's note: Copernicus Publications remains neutral with regard to jurisdictional claims in published maps and institutional affiliations.
This article is part of the special issue “Experiments in Hydrology and Hydraulics”. It is not associated with a conference.
The authors thank Günther Schmid and Matthias Karner for assistance during fieldwork, as well as Florian Darmann, Sebastian Rath, Julia Wutzl, Thomas Brunner, and Matthias Konzett for their help with the experiments. We are grateful to the agricultural experimental station in Wieselburg (Landwirtschaftliche Bundesversuchswirtschaften GmbH) for the permission to take soil monoliths on their premises.
This research has been supported by the Amt der NÖ Landesregierung (grant no. K3-F-130/005-2019).
This paper was edited by Daniel Green and reviewed by Narryn Thaman and two anonymous referees.
Abrantes, J. R. C. B., Moruzzi, R. B., Silveira, A., and de Lima, J. L. M. P.: Comparison of thermal, salt and dye tracing to estimate shallow flow velocities: Novel triple-tracer approach, J. Hydrol., 557, 362–377, https://doi.org/10.1016/j.jhydrol.2017.12.048, 2018.
Allaire, S. A. and van Bochove, E.: Collecting large soil monoliths, Can. J. Soil Sci., 86, 885–896, https://doi.org/10.4141/S05-062, 2006.
Andersson, H., Bergström, L., Ulén, B., Djodjic, F., and Kirchmann, H.: The Role of Subsoil as a Source or Sink for Phosphorus Leaching, J. Environ. Qual., 44, 535–544, https://doi.org/10.2134/jeq2014.04.0186, 2015.
Belford, R. K.: Collection and evaluation of large soil monoliths for soil and crop studies, J. Soil Sci., 30, 363–373, https://doi.org/10.1111/j.1365-2389.1979.tb00993.x, 1979.
Boix-Fayos, C., Martínez-Mena, M., Arnau-Rosalén, E., Calvo-Cases, A., Castillo, V., and Albaladejo, J.: Measuring soil erosion by field plots: Understanding the sources of variation, Earth-Sci. Rev., 78, 267–285, https://doi.org/10.1016/j.earscirev.2006.05.005, 2006.
Bottinelli, N., Zhou, H., Boivin, P., Zhang, Z. B., Jouquet, P., Hartmann, C., and Peng, X.: Macropores generated during shrinkage in two paddy soils using X-ray micro-computed tomography, Geoderma, 265, 78–86, https://doi.org/10.1016/j.geoderma.2015.11.011, 2016.
Bryan, R. B. and Luk, S.-H.: Laboratory experiments on the variation of soil erosion under simulated rainfall, Geoderma, 26, 245–265, https://doi.org/10.1016/0016-7061(81)90023-9, 1981.
Darch, T., Carswell, A., Blackwell, M. S. A., Hawkins, J. M. B., Haygarth, P. M., and Chadwick, D.: Dissolved Phosphorus Retention in Buffer Strips: Influence of Slope and Soil Type, J. Environ. Qual., 44, 1216–1224, https://doi.org/10.2134/jeq2014.10.0440, 2015.
Douglas, J. T., Ritchie, R. M., Takken, I., Crawford, C. E., and Henshall, J. K.: Large Intact Soil Slabs for studying the Effects of Soil and Plant Properties on Surface Runoff, J. Agric. Eng. Res., 73, 395–401, https://doi.org/10.1006/jaer.1999.0433, 1999.
Green, D. L.: Modelling Geomorphic Systems: Scaled Physical Models, in: Geomorphological Techniques, edited by: Cook, S. J., Clarke, L. E., and Nield, J. M., Britisch Society for Geomorphology, London, UK, 1–17, ISSN 2047-0371, 2014.
Jégou, D., Brunotte, J., Rogasik, H., Capowiez, Y., Diestel, H., Schrader, S., and Cluzeau, D.: Impact of soil compaction on earthworm burrow systems using X-ray computed tomography: preliminary study, Eur. J. Soil Biol., 38, 329–336, https://doi.org/10.1016/S1164-5563(02)01148-2, 2002.
Johnson, D. W., Walker, R. F., and Ball, J. T.: Lessons from Lysimeters: Soil N Release from Disturbance Compromises Controlled Environment Study, Ecol. Appl., 5, 395–400, https://doi.org/10.2307/1942030, 1995.
Katagi, T.: Soil Column Leaching of Pesticides, in: Reviews of Environmental Contamination and Toxicology, Vol. 221, edited by: Whitacre, D. M., Springer, New York, NY, 1–105, https://doi.org/10.1007/978-1-4614-4448-0_1, 2013.
Kuhn, N. J., Greenwood, P., and Fister, W.: Chapter 5.1 – Use of Field Experiments in Soil Erosion Research, vol. 18, edited by: Thornbush, M. J., Allen, C. D., and Fitzpatrick, F. A., Elsevier, 175–200, https://doi.org/10.1016/B978-0-444-63402-3.00011-X, 2014.
Lamandé, M., Labouriau, R., Holmstrup, M., Torp, S. B., Greve, M. H., Heckrath, G., Iversen, B. V., de Jonge, L. W., Moldrup, P., and Jacobsen, O. H.: Density of macropores as related to soil and earthworm community parameters in cultivated grasslands, Geoderma, 162, 319–326, https://doi.org/10.1016/j.geoderma.2011.03.004, 2011.
Lewis, J. and Sjöstrom, J.: Optimizing the experimental design of soil columns in saturated and unsaturated transport experiments, J. Contam. Hydrol., 115, 1–13, https://doi.org/10.1016/j.jconhyd.2010.04.001, 2010.
Luk, S. and Morgan, C.: Spatial variations of rainwash and runoff within apparently homogeneous areas, Catena, 8, 383–402, https://doi.org/10.1016/S0341-8162(81)80025-2, 1981.
Menta, C.: Soil Fauna Diversity – Function, Soil Degradation, Biological Indices, Soil Restoration, in: Biodiversity Conservation and Utilization in a Diverse World, edited by: Lameed, G. A., IntechOpen, London, UK, https://doi.org/10.5772/51091, 2012.
Palmer, R. E., Meisinger, J. J., and Magette, W. L.: Undisturbed Soil-Columns for Lysimetry: I. Collection, Field Testing, and Construction, Appl. Eng. Agric., 27, 379–389, https://doi.org/10.13031/2013.37073, 2011.
Pankau, R. C., Schoonover, J. E., Williard, K. W. J., and Edwards, P. J.: Concentrated flow paths in riparian buffer zones of southern Illinois, Agroforest. Syst., 84, 191–205, https://doi.org/10.1007/s10457-011-9457-5, 2012.
Pires, L. F., Bacchi, O. O. S., and Reichardt, K.: Assessment of soil structure repair due to wetting and drying cycles through 2D tomographic image analysis, Soil Till. Res., 94, 537–545, https://doi.org/10.1016/j.still.2006.10.008, 2007.
Poorter, H., Fiorani, F., Pieruschka, R., Wojciechowski, T., van der Putten, W. H., Kleyer, M., Schurr, U., and Postma, J.: Pampered inside, pestered outside? Differences and similarities between plants growing in controlled conditions and in the field, New Phytol., 212, 838–855, https://doi.org/10.1111/nph.14243, 2016.
Prosser, R. S., Hoekstra, P. F., Gene, S., Truman, C., White, M., and Hanson, M. L.: A review of the effectiveness of vegetated buffers to mitigate pesticide and nutrient transport into surface waters from agricultural areas, J. Environ. Manage., 261, 110210, https://doi.org/10.1016/j.jenvman.2020.110210, 2020.
Ramler, D., Stutter, M., Weigelhofer, G., Quinton, J. N., Hood-Nowotny, R., and Strauss, P.: Keeping Up with Phosphorus Dynamics: Overdue Conceptual Changes in Vegetative Filter Strip Research and Management, Front. Environ. Sci., 10, 1–8, https://doi.org/10.3389/fenvs.2022.764333, 2022.
Reid, K., Schneider, K., and McConkey, B.: Components of Phosphorus Loss From Agricultural Landscapes, and How to Incorporate Them Into Risk Assessment Tools, Front. Earth Sci., 6, 135, https://doi.org/10.3389/feart.2018.00135, 2018.
Roth, C. H. and Joschko, M.: A note on the reduction of runoff from crusted soils by earthworm burrows and artificial channels, Z. Pflanzenern. Bodenkd., 154, 101–105, https://doi.org/10.1002/jpln.19911540205, 1991.
Rüttimann, M., Schaub, D., Prasuhn, V., and Rüegg, W.: Measurement of runoff and soil erosion on regularly cultivated fields in Switzerland — some critical considerations, Catena, 25, 127–139, https://doi.org/10.1016/0341-8162(95)00005-D, 1995.
Sarmah, A. K., Pillai-McGarry, U., and McGarry, D.: Repair of the structure of a compacted Vertisol via wet/dry cycles, Soil Till. Res., 38, 17–33, https://doi.org/10.1016/0167-1987(96)01018-5, 1996.
Schneider, A. D., Marek, T. H., Ebeling, L. L., Howell, T. A., and Steiner, J. L.: Hydraulic Pulldown Procedure for Collecting Large Soil Monoliths, T. ASAE, 31, 1092–1097, https://doi.org/10.13031/2013.30828, 1988.
Sharpley, A. N.: Rainfall Frequency and Nitrogen and Phosphorus Runoff from Soil Amended with Poultry Litter, J. Environ. Qual., 26, 1127–1132, https://doi.org/10.2134/jeq1997.00472425002600040026x, 1997.
Singh, G., Kaur, G., Williard, K., Schoonover, J., and Kang, J.: Monitoring of Water and Solute Transport in the Vadose Zone: A Review, Vadose Zone J., 17, 160058, https://doi.org/10.2136/vzj2016.07.0058, 2018.
Tiefenbacher, A., Weigelhofer, G., Klik, A., Mabit, L., Santner, J., Wenzel, W., and Strauss, P.: Antecedent soil moisture and rain intensity control pathways and quality of organic carbon exports from arable land, Catena, 202, 105297, https://doi.org/10.1016/j.catena.2021.105297, 2021.
Weiler, M. and Naef, F.: An experimental tracer study of the role of macropores in infiltration in grassland soils, Hydrol. Process., 17, 477–493, https://doi.org/10.1002/hyp.1136, 2003.