the Creative Commons Attribution 4.0 License.
the Creative Commons Attribution 4.0 License.
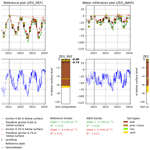
Effects of subsurface water infiltration systems on land movement dynamics in Dutch peat meadows
Sanneke van Asselen
Gilles Erkens
Christian Fritz
Rudi Hessel
Jan J. H. van den Akker
Large-scale drainage and cultivation of peat soils over the last centuries, occurring worldwide, have resulted in substantial CO2 emission and land subsidence caused by peat decomposition by microbial activity, shrinkage, and soil compaction. In addition, seasonal reversible vertical soil movement is caused by shrink and swell in the unsaturated zone and by poroelastic deformation in the saturated zone. To reduce CO2 emissions and land subsidence in drained peat soils, subsurface water infiltration systems (WISs) are expected to be a suitable measure. In this study, effects of WIS on seasonal vertical soil movements are evaluated, based on field measurements from five locations in Dutch peat meadows, for the years 2021 to 2023. First estimates of long-term land subsidence have also been made. At each study location, vertical soil movement has been measured using spirit leveling and extensometers in both a parcel with a WIS and a nearby reference parcel without any measure. Phreatic groundwater level fluctuations are found to induce soil volume decreases and increases in both the saturated and the unsaturated zone, which cause vertical land movement dynamics of up to 10 cm in the dry summer of 2022 at a location with a relatively thick (6 m) peat layer. Poroelastic deformation processes in the deeper saturated soil contribute substantially to surface-level movement and are largely reversible for the relatively short time period considered in this study. In peat meadows, subsurface water infiltration systems, if correctly applied, reduce seasonal vertical soil movements while (potentially) reducing soils' resilience to drought-induced volume losses. Seasonal vertical soil surface dynamics are about an order of magnitude higher than longer-term (years to decades) land subsidence rates, which are commonly of the order of millimeters per year in the Dutch drained peat areas as is supported by estimates of land subsidence from this study. Therefore, multiyear data series are needed to filter out variations in seasonal dynamics, which are mainly introduced by annual variations in weather conditions, and more accurately estimate long-term land subsidence.
- Article
(11386 KB) - Full-text XML
- BibTeX
- EndNote
Many coastal plains worldwide contain abundant peat in the subsurface that has formed over millennia under conditions of decelerating Holocene sea level rise (e.g., Stanley and Warne, 1994; Törnqvist et al., 2008; Drexler et al., 2009; van Asselen, 2011). At present, many coastal areas are densely populated (e.g., Neumann et al., 2015). Human activities, in particular drainage for land reclamation, have resulted in large-scale ending of peat formation in peat-rich coastal plains worldwide.
In the Dutch coastal peatlands, for example, drainage using ditches and artificial lowering of the phreatic groundwater table in peat soils already started about 1000 years ago to make the land suitable for agriculture. This resulted in increased peat decomposition by biogeochemical (oxidation) processes, resulting in CO2 emission and land subsidence due to loss of soil volume (Erkens et al., 2016) and an increase in bulk density due to loss of the fibric structure by humification. Lowered phreatic groundwater levels may also lead to land subsidence by irreversible soil shrinkage above and soil compaction below the phreatic groundwater level. Shrinkage is caused by suction forces that develop when the soil dries due to drainage and evapotranspiration as well as water extraction by roots. Soil compaction is induced by loading and/or structurally lowered pore water pressure following drainage, which increases the effective stress (Terzaghi, 1943; van Asselen et al., 2009, and references therein). In response to land subsidence following drainage, ditch water levels and therewith phreatic groundwater levels were lowered repeatedly in historical times to prevent the soil from becoming too wet for agricultural activities. This caused additional and (still) ongoing land subsidence. Despite drainage, most reclaimed peatlands have become too wet for crop growth and are therefore at present used as pastures for dairy farming.
Land subsidence due to peat compaction and decomposition is a slow and complex process taking place over long periods (many years; e.g., van Asselen et al., 2009). On shorter (days to months) timescales, reversible soil vertical movements commonly take place. In the unsaturated soil zone, these movements are especially caused by shrinkage and swelling of the soil as a result of periodic changes in soil suction forces driven by annual variations in evapotranspiration and precipitation, causing soil moisture changes. In the saturated soil, periodic variations in pore water pressures may cause seasonal poroelastic deformation, causing vertical soil movements. At one of the study sites of this research, preliminary results indicated short-term vertical surface-level movements of up to ∼ 4 cm (van Asselen et al., 2020). Irreversible land subsidence may also be affected by changes in rainfall patterns, which can significantly impact the water table levels and soil moisture profiles within peatlands. Changes in temperature may affect the rate of peat decomposition. Warmer temperatures accelerate the decomposition process, leading to a loss of peat's ability to support itself and contribute to subsidence. Conversely, cooler temperatures will slow down decomposition, potentially stabilizing the peatland temporarily. Climate change also influences vegetation cover, which impacts moisture levels of the soil through plant evapotranspiration.
Land subsidence in low-lying coastal areas may significantly contribute to relative sea level rise and increase the risk and impact of flooding (e.g., Ericson et al., 2006; Syvitski, 2008; Nicholls et al., 2021). Land subsidence also leads to (high costs related to) damage to buildings and infrastructure and may cause salinization (e.g., Van den Born et al., 2016). Also, short-term (seasonal) vertical movements may lead to damage and increased flood/inundation risks. In addition, land subsidence due to peat decomposition is linked to CO2 emissions. Over the last years, measures have been implemented that aim at reducing CO2 emissions from peatlands. It is not widely studied how these measures change land subsidence processes. Quantifying seasonal vertical movements and land subsidence in different environmental settings is needed to determine the relative contribution of different processes to total deformation and assess the proportions of vertical movements that are reversible and irreversible. This will lead to increased understanding of soil deformation processes and their main drivers, which is needed to be able to take effective measures to reduce land subsidence and CO2 emissions in drained peat meadows.
Peat decomposition and compaction may be reduced by rewetting peat soils; increased anaerobic conditions reduce microbiological activity, while higher pore water pressures in more saturated soils reduce the effective stress, thereby reducing or halting compaction processes. Cultivated peat soils could be completely rewetted and transformed into natural peatland, thereby losing their agricultural function. To maintain the agricultural land use function, which is often desirable for economic and cultural reasons, excessive lowering of the phreatic groundwater level in summer may be limited by installing subsurface water infiltration systems (WISs; Van den Akker et al., 2010; Querner et al., 2012). Drainpipes are brought into the soil at a depth of about 20 cm below ditch water level, which stimulates water infiltration from the ditch into the peat soils in dry periods with high evapotranspiration. To actively maintain and steer the phreatic groundwater level at a desired depth, independent of the ditch water level, drains may be connected to a reservoir with a pumping system (in this paper referred to as an active WIS; i.e., AWIS). Systems that are directly connected to a ditch, without a reservoir, are referred to as a passive WIS (PWIS).
The main aim of this paper is to assess effects of WIS on seasonal vertical soil movement in drained peat soils based on analysis of elevation changes obtained by spirit leveling and extensometers, groundwater level measurements, and subsurface lithological composition. In this study we focus on seasonal vertical soil movements since the time series are relatively short to make reliable estimates of longer-term (multiyear) land subsidence. Still, we include first estimates of long-term subsidence rates and evaluate effects of WIS on longer-term land subsidence. The research is part of the Netherlands Research Programme on Greenhouse Gas Dynamics in Peatlands and Organic Soils (NOBV). Data were collected in the period from mid-2020 to 2023 from five study sites distributed over the Dutch peatland area. For one of these locations data from 2019 were available. Field measurements are vital to monitor the efficiency and applicability of WIS under different environmental circumstances and possibly optimize these systems in order to stop or reduce land subsidence and CO2 emissions from drained peat soils (Aben et al., 2024).
The five study sites are presented in Fig. 1. At these locations, one field plot is situated in a WIS parcel and another field plot is situated in a nearby reference parcel without such a system but with similar environmental conditions.
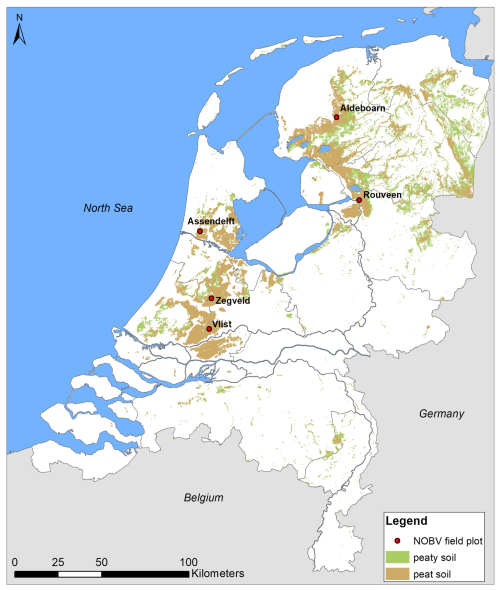
Figure 1Map of organic soils in the Netherlands and locations of paired field plots, each consisting of a WIS parcel and a nearby reference parcel. Source topographical map: TNO (2019). The peat and peaty soil map is derived from Brouwer et al. (2021).
At each study location, elevation changes have been monitored in both the WIS and reference parcel using two methods: spirit leveling and extensometry (see Sect. 2.1 and 2.2, respectively). In addition, groundwater and ditch water levels are monitored at each location (van Asselen et al., 2023). Also, the subsurface has been investigated by hand corings using Edelman and gouge augers and, at all locations except for Rouveen, also by a cone penetration test (CPT; Erkens et al., 2019). In general, the subsurface at all study sites consists of Holocene peat and clay layers overlying Pleistocene sandy deposits. Details of the groundwater system and subsurface composition at each study location are described in Sect. 2.3. In this study, estimates of the organic matter content mentioned in Sect. 3 section are derived from field observations from experienced geologists and soil scientists and from loss on ignition laboratory tests (LOI = ((dry weight − ashed weight)dry weight) × 100 %); see Heiri et al., 2001).
2.1 Spirit leveling
Spirit leveling is performed four times a year along fixed transects perpendicular to and in the longitudinal direction of a parcel relative to a local benchmark (a steel tube) that has been placed in firm Pleistocene sand underlying the soft Holocene peat and clay sequence. The elevation of this benchmark, relative to the Dutch Ordnance Datum (NAP), has been measured by spirit leveling to a nearby official NAP benchmark, which are regularly checked for stability. At each location, three to five transects of several tens of meters in length are measured per parcel, commonly using a measuring interval of 2 m. Measurements in ditches and on ditch banks are excluded from analyses in this study. The locations (x–y coordinates) of the beginning and end points of the transects are determined in each field campaign using an RTK-GNSS device to ensure leveling measurements are performed at the same locations. In Aldeboarn, the distance between two measurement points ranges from 2 to 7.5 m, and measuring points were marked belowground to allow for replication in time. The configuration of transects at the five study areas is presented in Sect. 2.3. Surface elevation is measured in winter (January), spring (April), summer (July), and autumn (October). This technique measures surface elevation with millimeter-scale accuracy. It should be taken into account that leveling results presented in this study are averages of ∼ 100 surface-level measurements on a parcel. Moreover, the surface level of a peat meadow is irregular due to, e.g., vegetation growth and trampling by cattle, and vertical soil movement may be spatially variable due to, e.g., variations in subsurface composition and local hydrology. Consequently, the standard deviation of calculated average elevation changes (relative to the first measurement; see Sect. 2.4) is commonly of the order of 10 to 20 mm.
2.2 Extensometry
The extensometers used in this study are specifically designed for high-resolution measurement of the vertical movement of multiple subsurface levels in soft peat and clay soils at millimeter accuracy. At each of the five study sites, an extensometer has been installed in both the WIS and the reference parcel. Each extensometer measures the vertical displacement at five anchor levels relative to a reference (massive cone) anchor fixed in a firm and stable sandy layer below the soft soil sequence (Fig. 2). The reference anchor is assumed to be stable. The depth of the firm sand has at most locations been determined based on the results of the CPT, which shows at what depth the resistance and friction of the soil significantly increases, indicating the occurrence of firm sand. By calculating the deformation of the soil layer between two anchors based on their vertical displacements, the contribution of different soil layers to total surface deformation in time may be determined (Fig. 2).
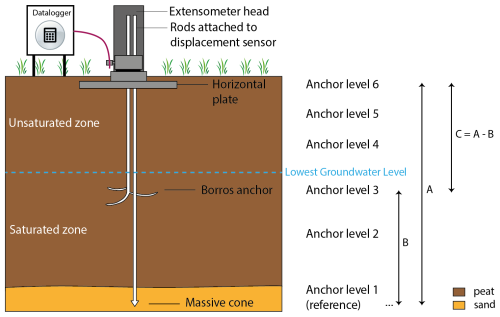
Figure 2Schematic representation of an extensometer setup. Deformation of soil layers may be calculated based on elevation changes of individual anchor levels; e.g., in the example above, the deformation of layer C is the elevation change of anchor level 6 (A; relative to the reference anchor 1) minus the elevation change of anchor level 3 (B; relative to the reference anchor 1).
Different types of anchors are used (Fig. 2).
-
Anchor level 1 is a massive cone, and this is the reference level. The anchor is installed using a cone penetration test vehicle. The bottom of the borehole that was made to install this anchor has been filled with grout. This was done to prevent connection from being created between the groundwater in the Holocene and the Pleistocene sequences, which may have different hydraulic heads. In Rouveen, the reference anchor has been installed by driving the (massive cone) anchor into the soil using a manual pile driver.
-
Anchor levels 2 to 4 are Borros-type anchors, consisting of three prongs that are firmly embedded in the soft soil. First, a small borehole is drilled using a gouge auger. Next, the Borros anchor is brought to the desired depth, after which the prongs are extended using hydraulic pressure. Fully extended, the prongs extend circa 150 mm into the soil.
-
Anchor level 5 is installed at a depth of circa 40 cm below the surface, which is too shallow for a Borros anchor. Instead, a horizontal steel strip is used as an anchor, which is brought into the undisturbed ground via a small trench.
-
Anchor level 6 is a square perforated stainless-steel plate (0.5 × 0.5 m, 8 mm perforation, about 40 % open area) that is dug into the soil at a depth of circa 5 cm, thereby largely representing surface-level vertical dynamics. The top centimeters of soil are removed before installation and put back on top of the plate after installation. The plate is installed at ca. 5 cm depth to prevent measuring vertical movement caused by especially vegetation growth and to ensure that the vertical movement measured can be attributed to soil deformation.
All anchors are connected to the extensometer head, which is fixed to the perforated stainless-steel plate (anchor level 6) with screwed stainless-steel rods (Fig. 3). The rods are protected by a ribbed tube, limiting friction of the (deforming) soil on the rods. In the extensometer head each rod is connected to the displacement sensor, which is either a vibrating wire displacement transducer or linear potentiometer. Because of limited available space in the extensometer head, this setup has a maximum of five anchor levels. The displacement sensors are connected to a datalogger next to the extensometer (Fig. 3). In this study, measurements are recorded hourly. In the WIS parcel, the extensometer is installed halfway between two drains; in the reference parcel the extensometer is installed at about one-third of the parcel width.
2.3 Study sites
2.3.1 Aldeboarn
In Aldeboarn, a PWIS was installed in 2016. In the summer following the installation of the PWIS, ditch water levels were raised from 80 to about 40 cm below the surface level of the field site (Fritz et al., 2021). Vertical elevation (change) has been measured using leveling and extensometry since mid-2020 (Fig. 4). Drains are installed between 70 and 80 cm depth in the longitudinal direction of the parcel. The distance between drains is ∼ 6 m. In Aldeboarn, a HAKLAM (in Dutch: “Hoog Als het Kan, Laag Als het Moet”, translating into “high if possible, low if necessary”) water level management has been applied. This means that the polder water level (and therefore the ditch water level of the reference plot) is set high (∼ 45 cm below the surface level) during dry periods and low if necessary for agricultural practice, such as for early growing season fertilization and/or the harvest of the last grass cut, which require sufficient load-bearing capacity of the peat soil. The ditch water level in the reference parcel fluctuated between ∼ 45 cm below the surface level in the summer of 2020 and ∼ 90 cm below the surface level in the first months of 2022 (van Asselen et al., 2023). In the PWIS parcel, the ditch water level has been fixed at ∼ 45 cm below the surface level since the growing season of 2022. Before that time, the ditch water level fluctuated between ∼ 40 and ∼ 80 cm below the surface level (van Asselen et al., 2023). Unfortunately, a reliable measurement of the hydraulic head in the sand layer below the peat layer is lacking at Aldeboarn, but the hydrological model LHM4.1 (LHM, 2023) indicates that on average this site is neutral (no significant seepage or infiltration). Further information about the groundwater measurements and the PWIS system, as well as how it is connected to the ditches, is provided by van Asselen et al. (2023).
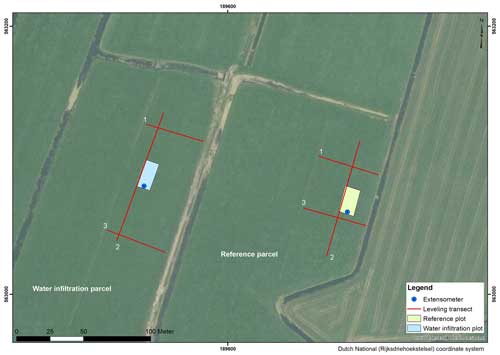
Figure 4Position of field plots, spirit leveling transects, and extensometers in the reference and PWIS parcel at Aldeboarn.
The subsurface in Aldeboarn is generally characterized by clay on peat on detritus on sand (see also soil profiles at extensometer locations in Table 1 and Erkens et al., 2019). The top clay layer is 0.25 to 0.65 m thick, deposited in a marine environment. The marine clay is often stiff and has an organic matter content (i.e., LOI) of about 15 %. Peat fragments may occur in the clay layer due to plowing (Fritz et al., 2021). Below the clay layer, to a maximum depth of 2.1 m below the surface, the subsurface consists of oligotrophic peat, containing remains of Sphagnum mosses, Eriophorum, and heather. The drained peat is strongly decomposed and amorphous, usually down to 65 cm depth and up to a depth of at maximum 0.95 m. At the transition to underlying Pleistocene sand, an amorphous sandy detritus layer 0.05 to 0.20 m thick is present. The top few meters of the Pleistocene deposits consist of an alternation of sandy and clayey layers. Firm sand, in which the reference anchor of the extensometer is installed, is encountered at a depth of about 7 to 8 m below the surface.
The x, y, and z coordinates and anchor depths of the extensometers in Aldeboarn are given in Table 1.
Table 1Positions and anchor depths of the extensometers in Aldeboarn. x and y coordinates are given in the Dutch National Grid (Rijksdriehoekstelsel). z (surface elevation) relative to the Dutch Ordnance Datum (NAP). The anchor level positions are also indicated in the soil profile (horizontal black lines; green: clay, brown: peat, yellow: sand, orange: sand and/or loam).
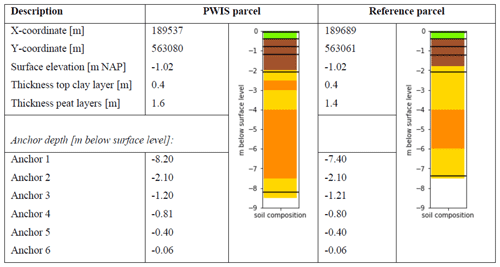
2.3.2 Rouveen
In Rouveen, drains are installed at circa 65 to 70 cm depth in the longitudinal direction of the parcel. The drain spacing is ∼ 8 m. The drains are connected to a submerged collector drain, which is directly connected to the ditch, making this a PWIS. Vertical soil movement has been measured by spirit leveling and extensometry since the end of 2018 (Fig. 5). During the measuring period, ditch water levels for both the reference and PWIS parcel fluctuated roughly between 40 and 50 cm below the surface level in summer and between 50 and 60 cm below the surface in winter (van Asselen et al., 2023). Rouveen is an upward seepage location (see van Asselen et al., 2023), and output of the hydrological model LHM4.1 indicates average upward seepage for the period 2011–2018 of about 0.4 mm d−1 at this location (LHM, 2023).
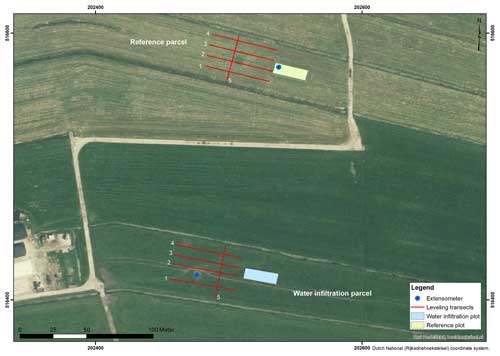
Figure 5Position of field plots, spirit leveling transects, and extensometers in the reference and PWIS parcel at Rouveen.
The subsurface in Rouveen is characterized by clay on peat on sand (see also soil profile figures at extensometer locations in Table 2 and Erkens et al., 2019). The top 0.05 to 0.10 m is composed of clayey peat with an organic matter content of ∼ 30 %; below this layer a generally stiff marine clay is found until ∼ 0.30 to ∼ 0.40 m depth with an organic matter content of ∼ 10 %. Below this clay layer peat occurs until a depth of 3.25 to 3.60 m below the surface, the depth at which sandy Pleistocene deposits occur. The top of the peat layer is strongly amorphous. Within 0.80 m below the surface a moss peat layer occurs of up to 0.20 m thick, containing sedge and wood remains. Below this layer a eutrophic sedge peat layer is found that may contain reed and wood remains. At some locations within the parcels, the peat is a bit clayey at a depth of 2.20 to 2.45 m below the surface.
Table 2Positions and anchor depths of the extensometers in Rouveen. x and y coordinates are given in the Dutch National Grid (Rijksdriehoekstelsel). z (surface elevation) relative to the Dutch Ordnance Datum (NAP). The anchor level positions are also indicated in the soil profile (horizontal black lines; green: clay, brown: peat, yellow: sand, orange: sand and/or loam).
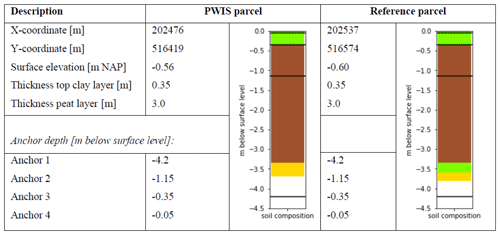
2.3.3 Assendelft
In Assendelft an active WIS was installed in 2017, aiming to keep the groundwater level at about 35 cm below the surface level. Drains are installed at circa 50 to 60 cm depth in the longitudinal direction of the parcel. The drain spacing is 4 m. Soil vertical movement has been measured using spirit leveling and extensometry since mid-2020 (Fig. 6). Ditch water levels for both the reference and AWIS parcels are mostly 20 to 45 cm below the surface level (van Asselen et al., 2023).
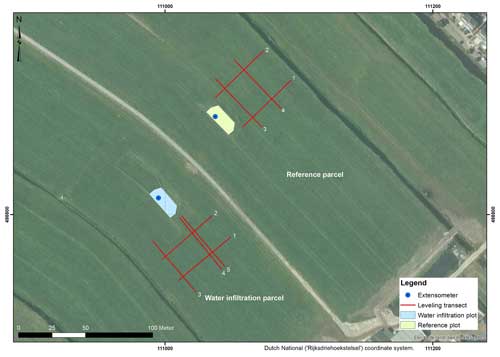
Figure 6Position of field plots, spirit leveling transects, and extensometers in the reference and AWIS parcel at Assendelft.
The hydraulic head in Assendelft (water pressure in sandy Pleistocene deposits at 17 m depth) is higher than the phreatic groundwater levels in the reference parcel (van Asselen et al., 2023). Upward seepage from this depth to the peat layer is, however, impeded by the thick (∼ 10 m) marine (clay, loam, and sand) deposits in between the peat and Pleistocene sand layers. Groundwater level measurements do indicate some upward seepage in dry periods at this location (van Asselen et al., 2023). Also, the hydrological model LHM4.1 (LHM, 2023) indicates some upward seepage at this location (∼ 0.1 mm d−1 on average for the period 2011–2018).
The Holocene sequence in Assendelft is about 16.5 m thick. In general, about 2 m of eutrophic reed–sedge peat is found on top of about ∼ 14 m of marine clayey and sandy deposits on ∼ 0.5 m of basal peat (see also soil profiles at extensometer locations in Table 3 and Erkens et al., 2019). The organic matter content (LOI) in the top 20 to 30 cm varies roughly between 10 % and 40 %. This layer has consequently been classified as either an organic clay or a clayey peat. Below the basal peat layer, Pleistocene sandy deposits occur at a depth of 16.5 m below the surface.
For x, y, and z coordinates and anchor depths of the extensometers in Assendelft see Table 3.
Table 3Positions and anchor depths of the extensometers in Assendelft. x and y coordinates are in the Dutch National Grid (Rijksdriehoekstelsel). z (surface elevation) relative to the Dutch Ordnance Datum (NAP). The anchor level positions are also indicated in the soil profile (horizontal black lines; green: clay, brown: peat, yellow: sand, orange: sand and loam).
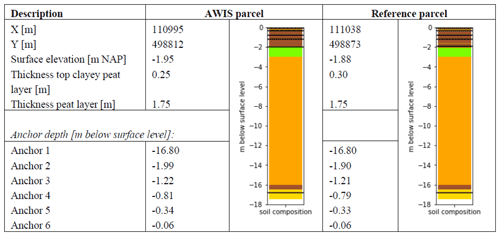
2.3.4 Zegveld
In Zegveld multiple measures are investigated: both active and passive water infiltration systems in combination with either relatively high or low ditch water levels (Fig. 7). Drains were installed in 2016 at about 70–75 cm depth in the longitudinal direction of the parcels. The drain spacing is 6 m. At parcel 16, ditch water levels are relatively low at ∼ 55 cm below the surface level. The target groundwater level of AWIS 16 is 40 cm below the surface level. At parcels 11 and 13 ditch water levels are kept relatively high at ∼ 25 to 30 cm below the surface level. The target groundwater level of AWIS 11 is 25 to 30 cm below the surface level in summer and 35 to 40 cm below the surface level in winter.
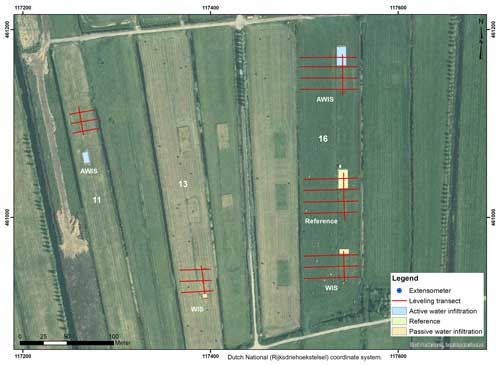
Figure 7Position of field plots, spirit leveling transects, and extensometers in the reference and water infiltration parcels at Zegveld. In parcel number 16 ditch water levels are relatively low (around 55 cm below the surface), and in parcel numbers 11 and 13 ditch water levels are relatively high (around 25 cm below the surface).
In Zegveld, a reliable automatic monitoring well for measuring the hydraulic head in the sandy Pleistocene subsurface was lacking during the monitoring period. The hydrological model, LHM, indicates a nearly neutral situation at this location, with minor infiltration of −0.06 mm d−1 (LHM, 2023) on average for the period 2011–2018.
The Holocene soft soil sequence in Zegveld is 6.10 to 6.35 m thick and consists predominantly of peat (see also soil profiles at extensometer location in Table 4 and Erkens et al., 2019). The top of underlying Pleistocene deposits consists of eolian cover sands. The top ∼ 0.20 to ∼ 0.50 m of the peat layer consists of clayey amorphous peat, with an organic matter content varying roughly between 30 % and 40 %, further down gradually increasing to about 80 %. In parcel 16, a 10 to 15 cm thick anthropogenic organic layer may occur at the surface consisting of historically applied cow manure and material dredged from the ditches, with variable sand admixture and/or pottery and brick remains (waste materials). To a depth of about 3 m predominantly wood peat occurs; below, eutrophic reed–sedge peat dominates, locally intercalated by thin reed peat layers. At a depth of 4.30 to 5.10 m below the surface a clayey interval a few centimeters thick occurs. Below this layer, the peat layer contains Cladium mariscus remains. At the transition to the Pleistocene sand wood peat may occur. Firm Pleistocene sand occurs at a depth of about 9 m below the surface.
The x, y, and z coordinates and anchor depths of the extensometers in Zegveld are given in Table 4.
Table 4Positions and anchor depths of the extensometers in Zegveld. x and y coordinates are given in the Dutch National Grid (Rijksdriehoekstelsel). z (surface elevation) relative to the Dutch Ordnance Datum (NAP). The anchor level positions are also indicated in the soil profile (horizontal black lines; green: clay, greenish brown: clayey peat or peaty clay, brown: peat, yellow: sand, orange: sand and/or loam).
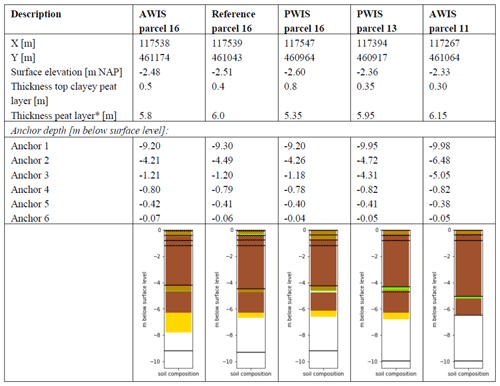
* including intercalated thin clayey intervals at about 4.5 m depth.
2.3.5 Vlist
In Vlist drains were installed in 2011 at circa 70 cm depth perpendicular to the longitudinal direction of the parcel, connected to one ditch, making this a passive WIS. The drain spacing is 6 m. For this study, elevation (change) has been measured by spirit leveling and extensometery since mid-2020 (Fig. 8). The ditch water level at both sides of the parcel has fluctuated between ∼ 45 and ∼ 60 cm below the surface level (van Asselen et al., 2023).
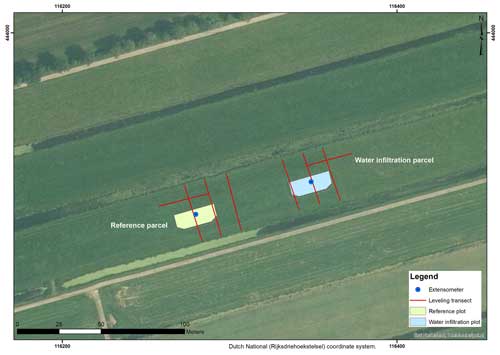
Figure 8Position of field plots, spirit leveling transects, and extensometers in the reference and PWIS parcel at Vlist.
The relatively short data series of the hydraulic head available for Vlist (measured since October 2022) shows a decreasing trend when the phreatic groundwater level is relatively low and an increasing trend when phreatic groundwater levels are high (van Asselen et al., 2023). This is an indication of some seasonal seepage/infiltration, although on average it is probably quite neutral, which is also indicated by the LHM (∼ −0.03 mm d−1 on average for the period 2011–2018; LHM, 2023).
The Holocene soft soil sequence in Vlist is 10 to 11 m thick and consists of an alternation of (fluvial) clay and peat layers generally several decimeters thick (see also soil profiles at extensometer locations in Table 5 and Erkens et al., 2019). Some of the clay layers contain sand. The top ∼ 0.40 m consists of humic clay and/or strongly clayey peat, with an organic matter content ranging between roughly 15 % and 35 % (highest at the top). Below the clayey top layer, a wood peat layer occurs, which may be a bit clayey. At a depth of about 2 m below the surface, a few decimeters of thick clay layer is found, below which eutrophic (sedge, reed, and/or wood) peat layers alternate with clay layers.
The x, y, and z coordinates and anchor depths of the extensometers in Vlist are given in Table 5.
Table 5Positions and anchor depths of the extensometers in Vlist. x and y coordinates are given in the Dutch National Grid (Rijksdriehoekstelsel). z (surface elevation) relative to the Dutch Ordnance Datum (NAP). The anchor level positions are also indicated in the soil profile (horizontal black lines; green: clay, brown: peat, yellow: sand, orange: sand and/or loam).
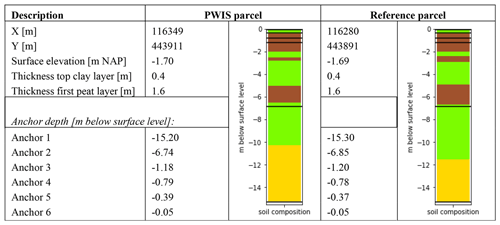
2.4 Data analysis
To get insight into seasonal vertical soil movement and long-term land subsidence, changes in elevation are calculated for each study site, for both spirit leveling and extensometer data, relative to the spirit leveling measurement day in January 2021 (time 00:00 for the hourly extensometer measurements). This reference was chosen because from this date onward, field measurements are available for all five locations. If available, measurements before this date are included, also relative to the leveling measurement in January 2021.
2.4.1 Spirit leveling data analysis
For each spirit leveling field campaign (four times a year), measured surface elevations relative to the Dutch Ordnance Datum (Normaal Amsterdams Peil; NAP) are averaged for both the WIS and reference parcel. After calculating the elevation changes, the yearly (2021 and 2022) vertical movement dynamics (D) for year x have been derived from the maximum (Hmax) and minimum (Hmin) elevation (relative to January 2021) within 1 year, calculated for the period 1 November year X−1 until 31 October year X using
In this way, the yearly vertical movement dynamics will in most cases represent seasonal subsidence going from the wet period during which the surface elevation is commonly highest (autumn and winter) to the dry period (spring and summer) when the surface elevation is commonly lowest.
2.4.2 Extensometer data analysis
In this study, extensometer anchors at approximately 0.05 and 0.80 m depth are included in the analysis as we focus on the effects of water infiltration systems on land movement dynamics and the contribution of the permanently saturated and unsaturated zone, respectively, below and above ca. 0.80 cm depth. For Rouveen, an anchor at 0.80 m depth was not available; hence, the anchor at 1.15 m depth was used instead. The current paper focuses on the effects of water infiltration systems on land movement dynamics, for which it is not necessary to include all anchors. In a separate paper (van Asselen et al., unpublished) focusing more on different processes contributing to land movement and subsidence and the relation to groundwater level dynamics and lithology, all anchor levels will be included. The (top) 0.05 m depth anchor is included because it best represents surface level, i.e., total deformation of the soft soil sequence, and therefore best compares with spirit leveling measurements of the surface level. The ∼ 0.80 m depth anchor was chosen to gain first insights into the relative contribution of the (predominantly) saturated soil below this level to vertical land surface movement. Elevation changes per anchor (hourly measurements) are calculated relative to the leveling field campaign day in January 2021 (at time 00:00). For each year, the relative contribution of the 0.80 or 1.15 m anchor to the 0.05 m (surface) anchor level was estimated based on the ratio of the dynamics of the 0.80 or 1.15 m anchor to the dynamics of the surface anchor level (×100 %).
In addition, for each leveling field measurement day, the average elevation changes of the 0.05 and 0.80 m depth anchors have been calculated, also relative to the leveling field campaign day in January 2021. These daily extensometer averages are used to compare measurements from both techniques.
The land movement data are plotted together with local high-resolution phreatic groundwater level measurements (for details see van Asselen et al., 2023). At WIS parcels, vertical land movement is compared with the phreatic groundwater dynamics from a monitoring well halfway between two drains (similar position as the extensometer). For the reference parcel, the phreatic groundwater level monitoring well closest to the extensometer has been used. At all sites, the ditch water level management did not change over the measuring period. The years considered in this study show different precipitation deficits: 70 mm in 2021, 233 in 2022, and 133 mm in 2023 (national averages), so one relatively wet year (2021), one dry year (2022), and one relatively standard year (2023).
To make first assessments of the long-term land subsidence rates, linear trend lines have been fitted on the yearly highest (winter) elevations (measurements in January and February) of the successive years of the extensometer surface anchor (at about −0.05 m depth) and the anchor at ∼ 80 or 115 cm depth. Note that these are first estimates and longer time series will result in more reliable estimates in the sense of higher R2. Hence, derived subsidence rates should be regarded with caution. Linear trend lines were used because we intend to estimate average subsidence rates for a specific time period, and moreover, the measurement period is still too short for investigating other types of fits. Also, another subsidence study that did have longer time series (a few decades) for the Zegveld site showed that a linear trend is adequate for assessing an average subsidence rate (Massop et al., 2024).
3.1 Aldeboarn
Both leveling and extensometer measurements demonstrate fewer vertical dynamics in the PWIS parcel compared to the reference parcel (3 to 20 mm less seasonal subsidence; Table 1; Fig. 9). In general, PWIS also resulted in less deep (maximum and average) groundwater levels in summer at this location (van Asselen et al., 2023; Fig. 9). The summer leveling measurement in 2021 is lacking but the extensometer data series shows relatively low summer subsidence for this period (21 mm; Table 1; Fig. 9), presumably related to the relatively wet summer of 2021 with relatively high phreatic groundwater levels. Results demonstrate a clear relation between vertical soil movement and groundwater dynamics (Fig. 9). For example, in the dry and warm summer of 2022, the phreatic groundwater level lowered substantially, which coincides with a relatively strong seasonal subsidence. The highest yearly vertical soil dynamics were measured in the reference parcel in 2022 (58 mm). Based on the extensometer data, we see a higher absolute effect on vertical dynamics of the PWIS in a dry year with relatively low groundwater levels (2022) compared to a wetter year with less deep groundwater levels (2021/2023). Furthermore, the extensometer data indicate that the contribution of the −0.80 m anchor to seasonal subsidence of the −0.06 m anchor is higher in the reference parcel than in the PWIS parcel. In general, changes in layer thickness relative to January 2021 have been a bit higher in the unsaturated layer (−0.06 to −0.80 m below the surface) compared to the saturated layer (>−0.80 cm below the surface), with less soil deformation of both layers in the WIS parcel compared to the reference parcel and an until now largely reversible movement in the saturated soil layer as can be seen from the fact that the change in the thickness of the saturated layer returns to approximately 0 each year, while the change in the thickness of the unsaturated layer does not (Fig. A1). This suggests that land subsidence has so far mainly been caused by peat decomposition and irreversible shrinkage in the top layer and not by peat compaction in the saturated layer. Fitted trend lines indicate average long-term land subsidence of 6 mm yr−1 in the reference parcel and 4 mm yr−1 in the PWIS parcel (0.06 anchor; Fig. 6).
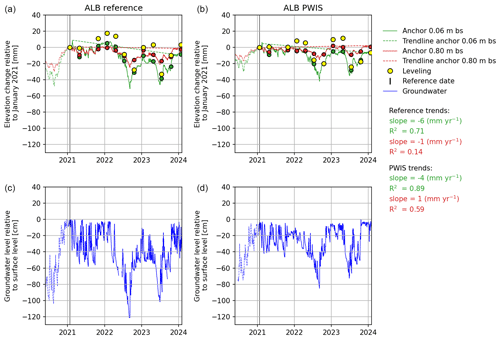
Figure 9Elevation changes relative to the leveling day in January 2021, indicated with the vertical black line, for the reference (ALB RF; a) and WIS (ALB PWIS; b) parcel in Aldeboarn based on spirit leveling (average of all measurement points per parcel) and extensometer measurements (surface anchor at 0.06 m depth in green and anchor at ∼ 0.80 m depth in red; daily averages for the leveling days are indicated with green and red dots, respectively). In blue the phreatic groundwater level is shown. Bs: below the surface. Data before the reference date are indicated with dotted lines.
Table 6Yearly vertical soil dynamics in millimeters for Aldeboarn based on leveling and extensometer measurements (anchors at 0.06 and 0.80 m depth). For leveling, the summer measurement is lacking for 2021, and therefore the spring measurement (end of April) has been used instead for calculating D. RF: reference parcel, Dif.: difference. Extensometer daily averages have been calculated for the days of the leveling measurements. The contribution of the 0.80 m anchor to the surface anchor (0.06 m depth) is given in the last column.
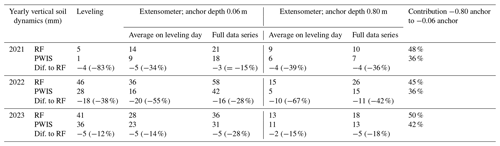
3.2 Rouveen
In general, the PWIS in Rouveen has resulted in lower phreatic groundwater levels compared to the reference parcel (van Asselen et al., 2023; Fig. 10). This undesired effect of the PWIS was attributed to substantial upward seepage in this area. Upward seepage causes relatively high phreatic groundwater levels, also in the summer season. The drains that were installed at a depth close to the deepest annual phreatic groundwater level have predominantly drained groundwater. Their role as infiltration drains has been minimal at this location. Field measurements do not show a clear effect of the PWIS on soil movement dynamics; in general, differences in dynamics between the reference and PWIS are relatively small and on several occasions in the opposite direction of what was expected (Table 7). The highest magnitude of vertical dynamics recorded at this location is 53 mm, measured by spirit leveling in the WIS parcel in 2021.
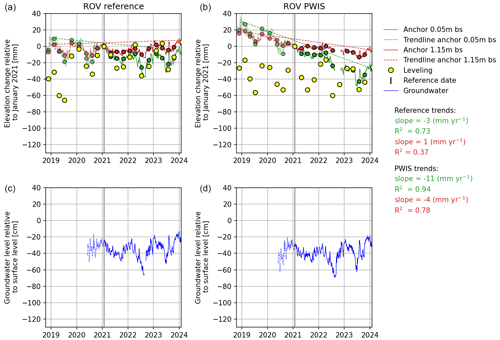
Figure 10Elevation changes relative to the leveling day in January 2021, indicated with the vertical black line, for the reference (ROV RF; a) and WIS (ROV PWIS; b) parcels in Rouveen based on spirit leveling and extensometer measurements (surface anchor at 0.05 m depth in green and anchor at 1.15 m depth in red; daily averages for the leveling days are indicated with green and red dots, respectively). In blue the phreatic groundwater level is shown. Data before the reference date are indicated with dotted lines or lighter colors.
Table 7Yearly vertical dynamics in millimeters for Rouveen based on leveling and extensometer measurements. RF: reference parcel, Dif.: difference. The extensometer daily average has been calculated for the day of the leveling measurement. The contribution of the 1.15 m anchor to the surface anchor (0.05 m depth) is given in the last column.
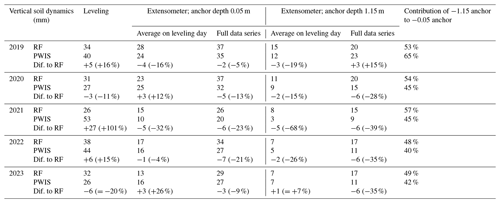
The linear trend lines indicate average land subsidence of 3 mm yr−1 (R2=0.73) for the reference parcel and 11 mm yr−1 (R2=0.94) for the PWIS parcel (Fig. 10). The linear trend line fitted on the 1.15 m anchor data indicates stable conditions (rising trend of 1 mm yr−1 with low R2 of 0.37) in the reference parcel and 4 mm yr−1 (R2=0.78) subsidence in the PWIS parcel. Hence, so far, the data suggest more subsidence in the PWIS parcel compared to the reference parcel, which could be explained by the lower phreatic groundwater levels measured in this parcel, causing increased peat decomposition and shrinkage in the unsaturated layer and peat compaction in the saturated layer (Fig. A2). In the reference parcel, land subsidence is predominantly caused by processes acting in the unsaturated soil layer above 1.15 m depth (Fig. A2).
3.3 Assendelft
The AWIS in Assendelft has resulted in higher summer phreatic groundwater levels (Fig. 11; see also van Asselen et al., 2023) and lower vertical soil dynamics in the AWIS parcel compared to the reference parcel. In general, periods with dropping groundwater levels coincide with soil subsidence, while periods with rising groundwater levels coincide with soil uplift. The effect of AWIS on both groundwater level and vertical soil dynamics (i.e., seasonal subsidence) is especially notable in the summers of 2022 and 2023 (Fig. 11; Table 3). The highest vertical magnitude in dynamics recorded is 79 mm based on the extensometer data series (−0.06 m anchor in 2022) in the reference parcel. The contribution of the −0.80 m anchor is relatively high (>50 %), especially in the wet year of 2021. In the reference parcel, soil deformation of the saturated soil layer below −0.80 m depth is higher than the unsaturated topsoil (Fig. A3). In the AWIS parcel, soil deformation is similar in both layers and is less than in the reference parcel. Soil deformation in the saturated layer is reversible so far, indicating that land subsidence (only in the reference parcel) is mainly caused by peat decomposition and shrinkage of the topsoil. Fitted trend lines indicate average long-term land subsidence of 7 mm yr−1 in the reference parcel and 1 mm yr−1 in the AWIS parcel (0.06 m anchor; Fig. 11).
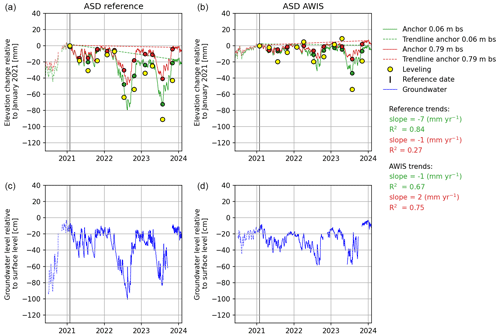
Figure 11Elevation changes relative to the leveling day in January 2021, indicated by the vertical black line, for the reference (ASD RF; a) and AWIS (ASD AWIS; b) parcels in Assendelft based on spirit leveling and extensometer measurements (surface anchor at 0.06 m depth in green and anchor at ∼ 0.80 m depth in red; daily averages for the leveling days are indicated with green and red dots, respectively). In blue the phreatic groundwater level is shown. Data before the reference date are indicated with dotted lines. Bs: below the surface.
3.4 Zegveld
In Zegveld, measurements took place in five fields in which different measures have been applied. In general, the different measures, i.e., type of WIS and high or low ditch water level, have resulted in less deep summer phreatic groundwater levels and lower yearly vertical soil dynamics compared to the reference parcel, as demonstrated by all types of elevation measurements applied at this study site (Table 4; Figs. 12; B1; Table B1; van Asselen et al., 2023). Again, results show a clear relation between vertical soil movement and groundwater dynamics (Fig. 12). The contribution of the −0.80 cm anchor is relatively high at Zegveld (>∼ 60 %) and is highest in the reference parcel. Lower vertical soil movement dynamics in the AWIS parcel compared to the reference parcel seem to be largely caused by less (poroelastic) deformation in the saturated soil below 0.80 m depth (Fig. A4). Fitted trend lines indicate average long-term land subsidence of 8 mm yr−1 in the reference parcel and 2 mm yr−1 in the PWIS parcel so far, and subsidence is mainly due to irreversible peat decomposition and shrinkage in the unsaturated zone at this location (Fig. A4).
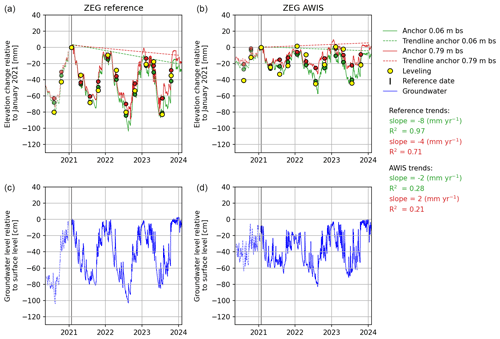
Figure 12Elevation changes relative to the leveling day in January 2021, indicated by the vertical black line, for two parcels in Zegveld (in parcel 16 with low ditch water level; ZEG REF, which is the reference parcel, and ZEG AWIS) based on spirit leveling and extensometer measurements (surface anchor at ∼ 0.05 m depth in green and anchor at ∼ 0.80 m depth in red; daily averages for the leveling days are indicated with green and red dots, respectively). In blue the phreatic groundwater level is shown. Data before the reference date are indicated with dotted lines. Bs: below the surface.
Yearly vertical soil dynamics are highest in the reference parcel. In the summer of 2022, the extensometer full data series recorded total dynamics of 98 mm in this parcel relative to the preceding winter. For most of the study sites, vertical dynamics were highest in the dry year of 2022. No clear relation between the type of measure and the calculated (reductions in) dynamics has been observed, but all measures showed different vertical dynamics compared to the reference parcel. Manual phreatic groundwater level measurements in 2019 and 2020 did show that summer phreatic groundwater levels were highest in the AWIS parcel, followed by the PWIS parcel, and were lowest in the reference parcel (Erkens et al., 2021).
3.5 Vlist
In general, measurements from Vlist indicate slightly higher summer phreatic groundwater levels (van Asselen et al., 2023) and slightly lower vertical dynamics in the PWIS parcel compared to the reference parcel (up to 10 mm lower vertical dynamics; Table 5; Fig. 13). The most pronounced effects are measured based on surface leveling. The highest fluctuation in vertical dynamics at this location is 47 mm, which was measured by spirit leveling in the reference parcel in 2022. The contribution of the −0.80 m anchor to the surface anchor is lowest in the dry year of 2022. The amount of soil deformation is fairly similar in the saturated and unsaturated layer of both the reference and PWIS parcels, where irreversible deformation mainly occurs in the unsaturated layer above ∼ 0.80 m depth (Fig. A5). Fitted trend lines indicate average long-term land subsidence 2 mm yr−1 in the reference parcel and 0 mm yr−1 in the PWIS parcel (Fig. 13).
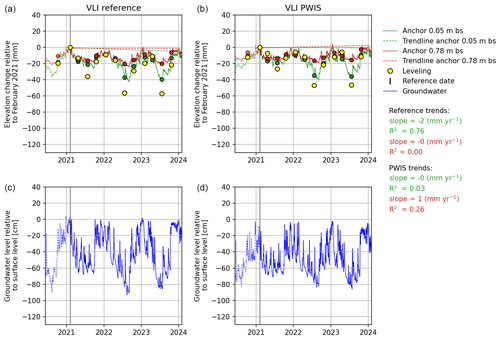
Figure 13Elevation changes relative to the leveling day in January 2021, indicated by the vertical black line, for the reference (VLI RF; left) and PWIS (VLI PWIS; right) parcels in Vlist based on spirit leveling and extensometer measurements (surface anchor at 0.05 m depth in green and anchor at ∼ 0.80 m depth in red; daily averages for the leveling days are indicated with green and red dots, respectively). In blue the phreatic groundwater level is shown. Data before the reference date are indicated with dotted lines. Bs: below the surface.
3.6 Main findings of vertical land movement measurements
At all locations except for Rouveen, the WIS has resulted in less lowering of the phreatic groundwater level in summer and, as a result of that, in reduced yearly vertical dynamics, i.e., seasonal soil subsidence. Compared to the reference parcels, the reduction of the yearly vertical soil dynamics is up to 74 % (measured at Assendelft in the summer of 2022 at the anchor level of ∼ 80 cm depth; Assendelft is also the location where the strongest effects on summer phreatic groundwater levels were measured; van Asselen et al., 2023). In absolute numbers, the AWIS in Assendelft resulted in up to 45 mm lower yearly vertical soil dynamics compared to the reference parcel. In Zegveld, AWIS resulted in up to 53 mm lower yearly vertical soil dynamics.
The high-resolution data series of vertical soil movement and phreatic groundwater level demonstrate that these two factors are strongly related, at different timescales, in the sense that a rise of the groundwater level results in upward soil movement and a drop of the groundwater level results in downward soil movement.
The highest yearly magnitude in vertical soil dynamics, i.e., seasonal subsidence, is 98 mm, which was recorded by the extensometer full data series in the summer of 2022 in the reference parcel of Zegveld.
Zegveld is also the location with the thickest peat layer (ca. 6 m thick) of all sites considered in this study. Firm sandy deposits are found at a depth of ca. 9 to 10 m. It is likely that a thicker peat layer results in larger vertical soil dynamics because a thicker layer is available, especially for poroelastic deformation. But, also in Assendelft, relatively high vertical soil dynamics have been observed. Here, the peat layer is less thick, ca. 2 m thick, but below this layer a ca. 10 m layer consisting of predominantly marine clay and sand is present. This thick Holocene layer is also subject to poroelastic deformation (see Fig. A3). The relation between lithology and soil dynamics will be further investigated in a follow-up paper (van Asselen et al., unpublished).
Deformation of the saturated subsurface (i.e., soil below ∼ 0.80 m depth or in the case of Rouveen below ∼ 1.15 m depth) considerably contributes to surface-level vertical movement. The contribution of deformation of the soil below this anchor level to surface subsidence is about 30 % to 95 % (based on extensometer measurements; Tables 6 to 10). At all study sites except for Rouveen, deformation of the saturated soil below 0.80 m depth is largely reversible so far, which suggests that long-term (irreversible) land subsidence is mainly caused by soil deformation processes in the unsaturated top layer (peat decomposition and irreversible shrinkage; see also Appendix A). However, longer time series are needed to exclude a contribution of deformation processes in the saturated peat in long-term subsidence. At the extensometer location in the PWIS parcel of Rouveen, where upward seepage water is drained, irreversible deformation of the saturated soil below 1.15 m depth has also taken place (Fig. A2).
Table 8Yearly vertical soil dynamics in millimeters for Assendelft based on leveling and extensometer measurements. RF: reference parcel, Dif.: difference. Extensometer daily averages have been calculated for the days of the leveling measurements. The contribution of the 0.80 m anchor to the surface anchor (0.06 m depth) is given in the last column.
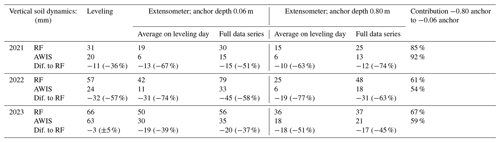
Table 9Yearly vertical soil dynamics in millimeters for Zegveld based on leveling and extensometer measurements. RF: reference parcel, Dif.: difference. Extensometer daily averages have been calculated for the days of the leveling measurements. The contribution of the 0.80 m anchor to the surface anchor (0.05 m depth) is given in the last column. In 2023 the vertical soil dynamics measured at 0.80 cm depth are larger than at 0.05 m depth, which indicates measurement uncertainties of the order of millimeters.
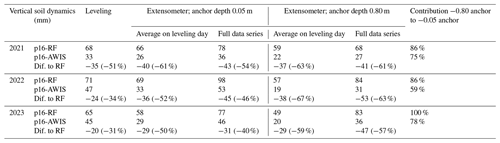
Table 10Yearly vertical soil dynamics in millimeters for Vlist based on leveling and extensometer measurements. RF: reference parcel, Dif.: difference. Extensometer daily averages have been calculated for the days of the leveling measurements. The standard deviation for elevation changes based on leveling is of the order of 10 to 20 mm. The contribution of the 0.80 m anchor to the surface anchor (0.05 m depth) is given in the last column.
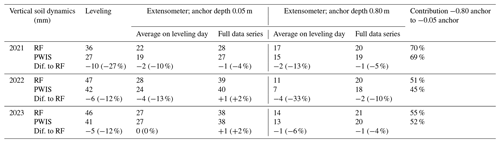
The yearly vertical dynamics derived from the daily averaged extensometer data are usually lower than the dynamics calculated from corresponding spirit leveling data (Tables 6 to 10). A likely explanation for this structural difference is deformation of the top ∼ 5 cm by shrinkage and swell, which is not measured by the extensometer. With spirit leveling, the surface level is measured.
The full extensometer data series, however, often yield higher vertical dynamics than those inferred from the spirit leveling (e.g., Aldeboarn, Assendelft, Zegveld). This suggests that quarterly measurements often miss most extreme elevations (minima and maxima) occurring within a year, which is indeed clearly noticeable when comparing the leveling data points (yellow) and the extensometer full data series (for example, Figs. 11 and 12).
Differences between leveling and extensometer surface-level measurements may also be caused by spatial variations in surface-level movement: the leveling data are spatial averages, while the extensometer measures at one location.
First estimates of long-term average land subsidence (top anchor) are in the range of 0 to −11 mm yr−1. Rates are lower in WIS parcels, except for Rouveen, where a multiyear subsidence rate of 3 mm yr−1 was estimated for the reference parcel and 11 mm yr−1 for the PWIS parcel. The unexpected higher subsidence rate estimated for the PWIS parcel at this upward seepage area is likely the result of lower summer groundwater levels due to increased drainage of seepage water.
The spirit leveling and extensometer techniques measure elevations at millimeter-scale accuracy. Uncertainties in the measured soil/surface elevation (changes) may especially arise from unstable reference levels, although these are all fixed in the firm sandy subsurface, making it unlikely that they moved over the study period (which is only a couple of years). The accuracy of the pressure sensor used to measure groundwater level is on the order of 1 cm, which has been tested in the laboratory. Additional uncertainties arise from deviations of the water level in a monitoring well compared to the actual phreatic groundwater level in the surrounding soil. However, because the measured groundwater level fluctuations are on the order of tens of centimeters and measured soil vertical movement is on the order of centimeters, it is unlikely that the mentioned uncertainties will affect the results and main findings of this study.
4.1 Effects of water infiltration systems on vertical soil movement
4.1.1 The relation between the phreatic groundwater level and soil deformation
The yearly groundwater level dynamics (range) and the deepest groundwater level are two hydrological characteristics that affect vertical soil movement through their influence on reversible and irreversible soil deformation processes. Our observations indeed indicate that phreatic groundwater level dynamics are a key factor controlling vertical movement, e.g., deformation, of peat soils (Figs. 9 to 13). Furthermore, for the five investigated study locations except for Rouveen, WIS resulted in less deep phreatic groundwater levels in summer periods (Figs. 9, 11 to 13; van Asselen et al., 2023) and therewith in lower yearly vertical soil movement.
The relation between the phreatic groundwater level and vertical soil movement is further indicated by the relation between the deepest yearly phreatic groundwater level and vertical dynamics of the shallowest extensometer anchor (Fig. 14). For most parcels, except for those in Rouveen, a positive relation between the deepest groundwater level and vertical dynamics, i.e., seasonal subsidence, is observed. Results of this study also demonstrate that phreatic groundwater level fluctuations at shorter (daily/weekly) timescales also affect vertical soil movement; a rise or drop of the groundwater level leads to an almost immediate response of soil deformation processes, respectively resulting in upward or downward soil movement (Figs. 9 to 13).
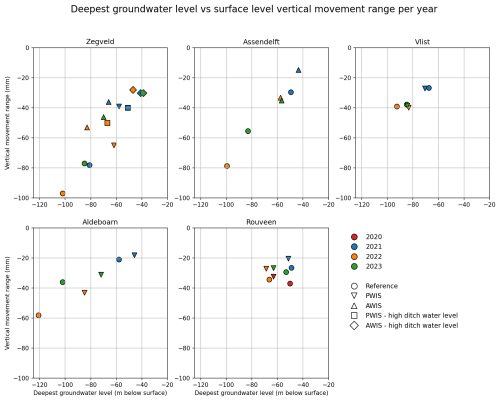
Figure 14Relation between the yearly vertical dynamics and deepest groundwater level for all parcels at all five locations.
In Rouveen, which is located in an area with upward seepage, the drains have unintendedly increased drainage, resulting in lower phreatic groundwater levels compared to the reference situation. Extensometer measurements indicate that this has resulted in more long-term subsidence in the PWIS parcel compared to the reference parcel, which also shows that the phreatic groundwater level dynamics are a key factor controlling vertical land movement.
4.1.2 Suppression of both unsaturated zone shrink–swell and saturated poroelastic deformation
The measurements presented in this study show that phreatic groundwater level dynamics affect reversible vertical soil movement, i.e., deformation, of the soil both above and below ∼ 0.80 m depth. Above this depth the soil is variably saturated and reversible soil deformation is in large part caused by shrink and swell processes as a result of variations in pore suction forces resulting from soil drying and wetting cycles. Less deep lowering of the phreatic groundwater level in summer as a result of WIS is expected to limit shrinkage of the unsaturated topsoil because, overall, the topsoil layer remains wetter and therewith suction forces remain lower compared to soils with lower summer groundwater levels. This is especially seen at Aldeboarn and Assendelft (see Sect. 3 and Appendix A).
Less deformation of the soil in the commonly saturated zone below ∼ 0.80 m depth may be explained by the effects of groundwater level dynamics on the effective stress in the subsurface. The effective stress, changes of which cause deformation of soil, is defined as the total stress (i.e., weight) minus the pore water pressure (Terzaghi, 1943). Fluctuations in the phreatic groundwater level cause temporal variations in pore water pressure and therewith effective stress, which causes (poroelastic) soil volume increases and decreases. Less deep summer groundwater levels, as a result of WIS, result in smaller variations of the pore water pressure and hence less soil deformation. This is especially clearly seen at Aldeboarn, Assendelft, and Zegveld (Appendix A). Reversible deformation in the saturated soil is referred to as poroelastic deformation (e.g., Kümpel, 1991). In Zegveld, the contribution of poroelastic deformation to surface-level vertical movement is relatively large, presumably due to the relatively thick (∼ 6 m) peat layer that is prone to deformation at this location. The total thickness of soil layers in which poroelastic deformation occurs is often much larger than the thickness of the unsaturated zone. So far, results suggest that soil deformation in the saturated soil layer is largely reversible (observed at all sites except the Rouveen PWIS plot).
Thus, results of this study demonstrate, as expected, that phreatic groundwater level dynamics and vertical soil movement dynamics are strongly related (Figs. 9 to 14). Commonly, a lowering of the groundwater level leads to downward soil movement (soil subsidence by shrinkage and poroelastic deformation), while a rise of the groundwater level leads to upward soil movement (by swell and poroelastic deformation). It is expected that this relation applies to all organic soils. Poroelastic deformation is commonly larger in organic soils than in clay soils. Shrinkage and swell properties may vary among different peat and clay soils (Peng et al., 2006).
4.1.3 Suppression of subsidence
It is expected that WIS will also reduce long-term subsidence through its effect on groundwater level dynamics, which affect multiple soil deformation processes. Firstly, if deep summer phreatic groundwater levels can be prevented by WIS and groundwater level dynamics are reduced, there will be fewer variations in pore water pressures and therewith effective stresses. This reduces the risk of irreversible soil compaction. In addition, the risk for irreversible shrinkage is expected to be lower because generally wetter soil conditions will lower the risk of severe drying of the soil. Moreover, soil moisture and temperature are main drivers of peat decomposition (Boonman et al., 2022). Less deep phreatic groundwater levels in warm summer are expected to lead to less loss of soil volume due to aerobic peat decomposition by microbial activity because oxygen can intrude less deeply into the soil, creating suboptimal (soil moisture) conditions for peat decomposition.
First estimates of long-term average subsidence rates vary between 2 and 8 mm yr−1 in the reference field sites and between 0 and 4 mm yr−1 in WIS field sites where WIS resulted in the desired effect of less deep groundwater levels in summer. For all sites except Rouveen, the estimated rate is lower in the WIS parcel compared to the reference parcel. In Rouveen, WIS resulted in deeper groundwater levels and a higher long-term average subsidence rate of 11 mm yr−1 (as opposed to 3 mm yr−1 at the Rouveen reference site). Reduced land subsidence rates in WIS parcels are seemingly largely caused by reduced peat decomposition and/or irreversible shrinkage in the unsaturated topsoil (Appendix A), though longer time series are needed to assess the long-term contribution of different subsidence processes more accurately. Estimated rates are of the same order of magnitude as subsidence rates in similar peat areas found in other Dutch (e.g., Schothorst, 1977; Beuving and Van den Akker, 1997; Massop et al., 2024) and international (Bloom, 1964; Haslett et al., 1998; Edwards, 2006; Fritz, 2006; Long et al., 2006; Törnqvist et al., 2008; Horton and Shennan, 2009) studies. Variations in both time and space in subsidence rates may be caused by variations thickness of the peat layer, peat type, peat decomposition rate, peat density, intensity of drainage, and climatic conditions (Egglesmann et al., 1993; van Asselen et al., 2009).
The higher subsidence rate in the PWIS parcel of Rouveen is presumably related to (unintendedly) deeper average phreatic groundwater levels in this parcel causing increased peat decomposition and compaction. This observation supports the existence of a positive relation between phreatic groundwater level depth and long-term land subsidence The effect of groundwater levels on long-term subsidence is also shown by Massop et al. (2024), who demonstrate that, based on long-term (circa 50 years) spirit level measurements of surface level and soil markers at different depths, long-term subsidence in a field with relatively high ditch water levels (and related higher phreatic groundwater levels) was less than in a field with relatively low ditch water levels (and related lower phreatic groundwater levels).
4.2 Spirit leveling versus extensometer technique
In this study, spirit leveling and extensometery have been used to assess vertical soil movement dynamics and multiyear land subsidence in peat meadow areas. Both field techniques can measure elevation changes at millimeter-scale precision. An important difference between these two techniques relates to their temporal and spatial applicability. An extensometer measures vertical soil movement at one location and therefore does not provide information on spatial variability of vertical soil movement within a parcel. The temporal resolution is high, however (hourly measurements), and soil movement is measured at various depths. This allows detailed analysis of land movement dynamics and subsurface deformation that relates these to other conditions like groundwater level dynamics and variations in subsurface composition. This is vital to understand and unravel different processes contributing to land movement dynamics and long-term subsidence. Spirit leveling has a higher spatial coverage, measuring along multiple transects, but the temporal resolution is commonly low (four times a year in this study, often less), which brings the risk of missing vertical dynamics (see also figures in Sect. 3). Consequently, in particular in the case of spirit leveling, long time series are essential, as demonstrated by, for example, the leveling time series of Rouveen that do not yet indicate a clear long-term trend with a low R2, while the extensometer measurements at this location do result in a subsiding trend with fairly high R2.
Indeed, our measurements demonstrate that the range derived from the high-resolution extensometer measurements is often higher than the range derived from spatially averaged spirit leveling data (see result sections). Dynamics derived from extensometer measurements averaged for the day of spirit leveling are, however, often lower compared to dynamics derived from spirit leveling; this may be due to deformation of the top ∼ 5 cm of soil between the surface level and the topmost extensometer anchor, which is not registered by the extensometer surface anchor. These differences should be carefully considered and taken into account when using these methods for measuring vertical soil movement.
The leveling and extensometer data series presented in this study show that yearly vertical soil dynamics, i.e., seasonal subsidence, of the surface is of the order of centimeters. The largest vertical dynamics of almost 10 cm were measured in an area with a relatively thick (6 m) peat layer in the dry and warm summer of 2022. This study also demonstrated that seasonal vertical soil movement is about an order of magnitude higher than long-term subsidence rates in drained peatlands (commonly millimeters per year). Therefore, multiyear data series are needed to filter out seasonal (variable) dynamics and estimate long-term subsidence. In this study, first estimates of long-term average subsidence rates based on linear fits on extensometer data series indicate subsidence rates of 2 to 8 mm yr−1 at the reference field sites and 0 to 4 mm yr−1 at WIS field sites, with variable R2 values. In Rouveen, where WIS resulted in deeper phreatic groundwater levels, a long-term average subsidence rate of 11 mm yr−1 was estimated. Longer data series are needed to make (more) reliable (with higher R2) estimates of land subsidence.
Results of this study demonstrate that short-term (daily to monthly) vertical soil movements are related to phreatic groundwater level fluctuations, which affect soil deformation processes in both the unsaturated and saturated zone. Water infiltration systems in peat meadows, if correctly applied, limit phreatic groundwater level lowering in summer periods and therewith also limit seasonal subsidence. Less deep summer phreatic groundwater levels cause less shrinkage in the unsaturated zone due to higher water content and lower suction forces. In the saturated zone, higher phreatic groundwater levels cause less poroelastic deformation because of reduced changes in pore water pressure and therewith effective stress. Poroelastic deformation of the saturated subsoil (below ∼ 0.80 m depth) may considerably contribute to surface-level movement, often more than processes in the unsaturated zone, especially if the subsurface is composed of a relatively thick peat layer. We found that poroelastic deformation in the saturated zone is largely reversible for the relatively short time period considered in this study, while soil deformation processes in the unsaturated zone are partly irreversible, which leads to long-term land subsidence. At longer timescales, irreversible deformation processes in the saturated soil could also contribute to total land subsidence (e.g., Massop et al., 2024).
Differences in the temporal and spatial resolution of the spirit leveling and extensometer techniques require careful consideration for developing land movement monitoring plans. Spirit leveling measurements give insight into the spatial variability of elevation and elevation changes within a parcel. This technique is especially useful for long-term (at least 10 years of measurements) application to determine average long-term subsidence of a parcel. Extensometer measurements are particularly useful for high-resolution monitoring of soil movement dynamics at different depths, which allows quantifying both short- and long-term soil movement and better understanding soil deformation processes in different soil layers as well as their relative contribution to surface-level movement.
Figures are given here for changes in the thickness, relative to the winter of 2021, of the unsaturated layer between ∼ 0.05 and ∼ 0.80 or 1.15 (for Rouveen) m depth and the saturated layer below ∼ 0.80 or 1.15 (for Rouveen) m depth for both the WIS and reference parcel for all five locations.
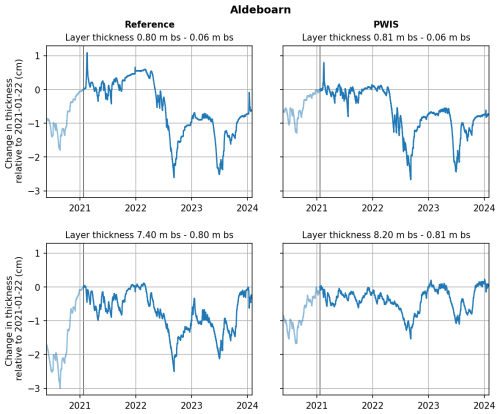
Figure A1Changes in layer thickness relative to the winter of 2021 of the unsaturated layer between ∼ 0.05 and ∼ 0.80 m depth and the saturated layer below ∼ 0.80 m depth for both the WIS and reference parcel at the Aldeboarn study site.
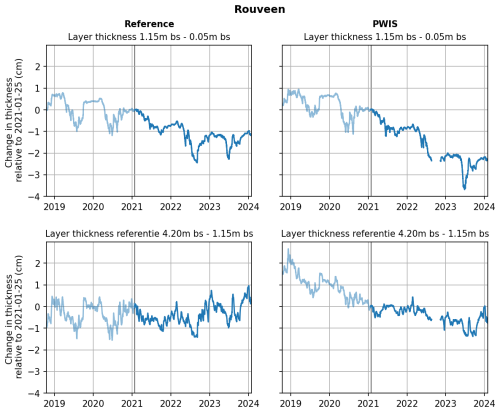
Figure A2Changes in layer thickness relative to the winter of 2021 of the unsaturated layer between ∼ 0.05 and ∼ 1.15 m depth and the saturated layer below ∼ 1.15 m depth for both the WIS and reference parcel at the Rouveen study site.
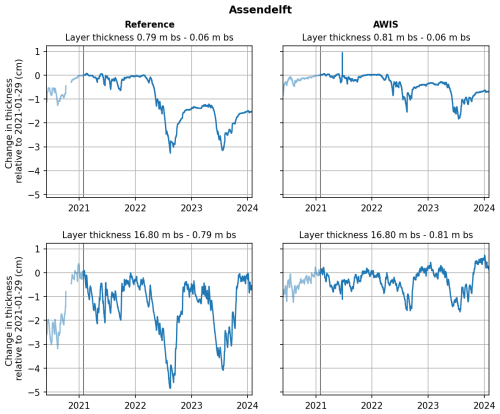
Figure A3Changes in layer thickness relative to the winter of 2021 of the unsaturated layer between ∼ 0.05 and ∼ 0.80 m depth and the saturated layer below ∼ 0.80 m depth for both the WIS and reference parcel at the Assendelft study site.
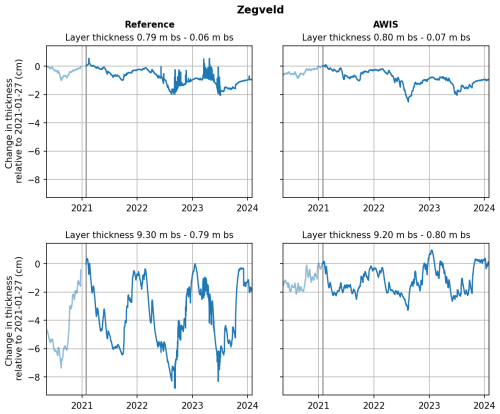
Figure A4Changes in layer thickness relative to the winter of 2021 of the unsaturated layer between ∼ 0.05 and ∼ 0.80 m depth and the saturated layer below ∼ 0.80 m depth for both the WIS and reference parcel at the Zegveld study site.
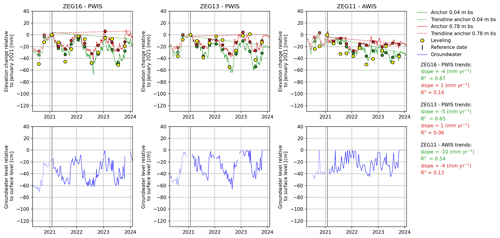
Figure B1Elevation changes relative to the leveling day in January 2021, indicated by the vertical black line, for three parcels in Zegveld (PWIS in parcel 16 with low ditch water level, PWIS in parcel 13 with high ditch water level, and ASIW in parcel 11 with variable ditch water level) based on spirit leveling and extensometer measurements (surface anchor at ∼ 0.05 m depth in green and anchor at ∼ 0.80 m depth in red; daily averages for the leveling days are indicated with green and red dots, respectively). In blue the phreatic groundwater level is shown, based on manual measurements. Data before the reference date are indicated with dotted lines. The standard deviation for elevation changes based on leveling is of the order of 10 to 20 mm. Bs: below the surface.
Table B1Yearly vertical soil movement ranges (maximum height minus minimum height) in millimeters for Zegveld based on leveling and extensometer measurements. RF: reference parcel, Dif.: difference. Extensometer daily averages have been calculated for the days of the leveling measurements. The contribution of the 0.80 m anchor to the surface anchor (0.05 m depth) at the moment of maximum yearly subsidence (minimum elevation relative to maximum elevation in the preceding winter) is given in the last column. Note that parcels 11 and 13 have different ditch water levels compared to parcel 16, so differences should be taken with caution (both ditchwater level and WIS influence differences compared to the reference situation).
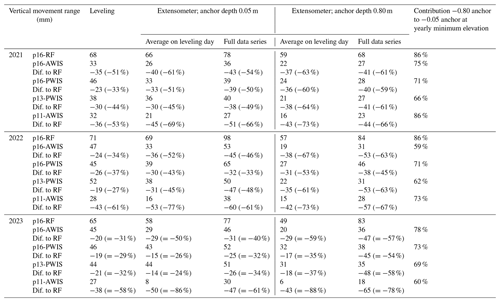
Data used in this research are described in the article. Raw data are stored at the Deltares Research Institute and the NOBV database and can be made available by contacting one of the authors of this article or via info@nobveenweiden.nl.
SvA performed all analyses and wrote and prepared the manuscript, with contributions from all authors and others (see Acknowledgements).
The contact author has declared that none of the authors has any competing interests.
Publisher’s note: Copernicus Publications remains neutral with regard to jurisdictional claims made in the text, published maps, institutional affiliations, or any other geographical representation in this paper. While Copernicus Publications makes every effort to include appropriate place names, the final responsibility lies with the authors.
We would like to thank Harry T. L. Massop, Paul A. Gerritsen, Stefan T. J. Weideveld, Oswin van der Scheer, Enno van Waardenberg, Dennis Peters, Kevin Mouthaan, Sannimari Käärmelahti, Peter Cruijsen, Roy Belderok, and Mark Stoetzer for all of their fieldwork-related activities. Siem Jansen and Jesse Reusen are thanked for their help with data analysis and visualization.
This research was carried out within the Netherlands Research Programme on Greenhouse Gas Dynamics in Peatlands and Organic Soils (NOBV; https://www.nobveenweiden.nl/en/, last access: 31 March 2025) and was cofunded by the WUR internal program KB34 Towards a Circular and Climate Neutral Society (2019–2022), project KB34-005-001 (Peat areas in new circular and climate-positive production systems).
This paper was edited by Serena Ceola and reviewed by two anonymous referees.
Aben, R. C. H., van de Craats, D., Boonman, J., Peeters, S. H., Vriend, B., Boonman, C. C. F., van der Velde, Y., Erkens, G., and van den Berg, M.: CO2 emissions of drained coastal peatlands in the Netherlands and potential emission reduction by water infiltration systems, Biogeosciences, 21, 4099–4118, https://doi.org/10.5194/bg-21-4099-2024, 2024.
Beuving, J. and Van den Akker, J. J. H.: Maaiveldsdaling van veengrasland bij twee slootpeilen in de polder Zegvelderbroek, Vijfentwintig jaar zakkingsmetingen op het ROC Zegveld, Rapport, vol. 377, DLO-Staring Centrum, Wageningen, https://edepot.wur.nl/302578 (last access: 1 April 2025), 1997 (in Dutch).
Bloom, A. L: Peat accumulation and compaction in a Connecticut coastal marsh, J. Sediment. Petrol., 34, 599–603, 1964.
Boonman, J., Hefting, M. M., van Huissteden, C. J. A., van den Berg, M., van Huissteden, J., Erkens, G., Melman, R., and van der Velde, Y.: Cutting peatland CO2 emissions with water management practices, Biogeosciences, 19, 5707–5727, https://doi.org/10.5194/bg-19-5707-2022, 2022.
Brouwer, F., Maas, G., Teuling, K., Harkema, T., and Verzandvoort, S.: Bodemkaart en Geomorfologische Kaart van Nederland: actualisatie 2020-2021 en toepassing; Deelgebieden Gelderse Vallei Zuid en – West en Veluwe-Zuid, Wettelijke Onderzoekstaken Natuur and Milieu, WOt, Technical Report 134, https://edepot.wur.nl/557455 (last access: 1 April 2025), 2021 (in Dutch).
Drexler, J. Z., de Fontaine, C. S., and Deverel, S. J.: The legacy of the wetland drainage on the remaining peat in the Sacramento – San Joaquin Delta, California, USA, Wetlands, 29, 372–386, 2009.
Edwards, R. J.: Mid to late Holocene sea-level change in southwest Britain and the influence of sediment compaction, Holocene, 16, 575–587, https://doi.org/10.1191/0959683606hl941rp, 2006.
Egglesmann, R., Heathwaite, A. L., Gross-Braukmann, G., Kuster, E., Naucke, W., Schich, M., and Schweikle, V.: Physical processes and properties of mires. In Heathwaite, A. L. and Gottlich, Kh, editors, Mires, process, exploration and conservation, John Wiley and Sons, Chichester, 171–262, ISBN 0-471-93353-8, 1993.
Ericson, J. P., Vörösmarty, C. J., Dingman, S. L., Ward, L. G., and Meybeck, M.: Effective sea-level rise and deltas: causes of change and human dimension implications, Global Planet. Change, 50, 63–82, 2006.
Erkens, G., Van der Meulen, M. J., and Middelkoop, H.: Double trouble: subsidence and CO2 respiration due to 1,000 years of Dutch coastal peatlands cultivation, Hydrogeol. J., 24, 551–568, https://doi.org/10.1007/s10040-016-1380-4, 2016.
Erkens, G., van Asselen, S., Hommes, S., Melman, R., Van Meerten, H., Van Essen, H., Van den Berg, M., Aben, F., Fritz, C., Hessel, R., Van de Craats, D., Massop, H., Gerritsen, P., Van den Akker, J., Van 't Hull, J., Velthof, G., Van de Velde, Y., Van Huissteden, K., Boonman, J., Lootens, R. Hefting, M., Keuskamp. J., Hutjes, R., Kruijt, B., and Franssen, W.: Nationaal Onderzoeksprogramma Broeikasgassen Veenweiden (NOBV), jaarrapportage 2019–2020, NOBV, http://www.nobveenweiden.nl (last access: 31 March 2025), 2019 (in Dutch).
Erkens, G., van Asselen, S., Hommes-Slag, S., Melman, R., Kooi, H., Van Essen, H., Van den Berg, M., Aben, R., Fritz, C., Boonman, C., Velthuis, M., Heuts, T., Nouta, R., Hessel, R., Van de Craats, D., Massop, H., Gerritsen, P., Van 't Hull, J., Velthof, G., Van den Akker, J., Van Houwelingen, K., Van der Velde, Y., Boonman, J., Lootens, R., Van Huissteden, K., Hefting, M., Hutjes, R., Kruijt, B., Harpenslager, S. F., Van Dijk, G., Van de Riet, B., and Smolders, F.: Nationaal Onderzoeksprogramma Broeikasgassen Veenweiden (NOBV) – Data-analyse 2020–2021, https://www.nobveenweiden.nl/wp-content/uploads/2021/11/NOBV-Data-analyse-2020-2021.pdf (last access: 31 March 2025), 2021 (in Dutch).
Fritz, C.: Surface oscillation in peatlands: How variable and important is it?, Master Degree thesis, The University of Waikato, https://researchcommons.waikato.ac.nz/entities/publication/c82efd30-cad8-473a-9f34-e2fb6045682f (last access: 1 April 2025), 2006.
Fritz, C., Weideveld, S., Velthuijs, M., and Van den Berg, M.: Broeikasgasuitstoot van Friese veenbodems – Kunnen onderwaterdrainage en infiltratie aan een duurzame emissiereductie bijdragen?, Nijmegen, 1–108 pp., https://www.veenweidefryslan.frl/uploads/assets/docs/broeikasgasuitstoot-van-friese-veenbodems-2021.pdf (last access: 31 March 2025), 2021 (in Dutch).
Haslett, S. K., Davies, P., Curr, R. H. F., Davies, C. F. C., Kennington, K., King, C. P., and Margetts, A. J.: Evaluating late-Holocene relative sea-level change in the Somerset Levels, southwest Britain, Holocene 8, 197–207, https://doi.org/10.1191/095968398669499299, 1998.
Heiri, O., Lotter, A. F., and Lemcke, G.: Loss on ignition as a method for estimating organic and carbonate content in sediments: reproducibility and comparability of results, J. Paleolimnol., 25, 101–110, 2001.
Horton, B. P. and Shennan, I.: Compaction of Holocene strata and the implications for relative sea-level change, Geology 37, 1083–1086, https://doi.org/10.1130/G30042A.1, 2009.
Kümpel, H. J.: Poroelasticity: parameters reviewed, Geophys. J. Int., 105, 783–799, 1991.
LHM: Landelijk Hydrologisch Model, https://data.nhi.nu (last access: 31 March 2025), 2023 (in Dutch).
Long, A. J., Waller, M. P., and Stupples, P.: Driving mechanisms of coastal change: Peat compaction and the destruction of late Holocene coastal wetlands, Mar. Geol., 225, 63–84, https://doi.org/10.1016/j.margeo.2005.09.004, 2006.
Massop, H., Hessel, R., Van den Akker, J., Gerritsen, P., and Gerritsen, F.: Monitoring long-term peat subsidence with subsidence platens in Zegveld, the Netherlands, Geoderma 450, 117039, https://doi.org/10.1016/j.geoderma.2024.117039, 2024.
Neumann, B., Vafeidis, A. T., Zimmermann, J., and Nicholls, R. J.: Future Coastal Population Growth and Exposure to Sea-Level Rise and Coastal Flooding – A Global Assessment, PLoS ONE, 10, e0118571, https://doi.org/10.1371/journal.pone.0118571, 2015.
Nicholls, R. J., Lincke, D., Hinkel, J., Brown, S., Vafeidis, A. T., Meyssignac, B., Hanson, S. E., Merkens, J.-L., and Fang, J.: A global analysis of subsidence, relative sea-level change and coastal flood exposure, Nat. Clim. Change, 11, 338–342, https://doi.org/10.1038/s41558-021-00993-z, 2021.
Peng, X., Horn, R., Peth, S., and Smucker, A.: Quantification of soil shrinkage in 2D by digital image processing of soil surface, Soil. Till. Res., 91, 173–180, https://doi.org/10.1016/j.still.2005.12.012, 2006.
Querner, E., Jansen, P., Van den Akker, J., and Kwakernaak, C.: Analysing water level strategies to reduce soil subsidence in Dutch peat meadows, J. Hydrol., 446, 59–69, 2012.
Schothorst, C. J.: Subsidence of low moor peat soils in the western Netherlands, Geoderma, 17, 265–291, 1977.
Stanley, D. J. and Warne, A. G.: Worldwide initiation of Holocene marine deltas by deceleration of sea-level rise, Science, 265, 228–231, https://doi.org/10.1126/science.265.5169.228, 1994.
Syvitski, J. P. M.: Deltas at risk, Sustain. Sci., 3, 23–32, https://doi.org/10.1007/s11625-008-0043-3, 2008.
Terzaghi, K.: Theoretical Soil Mechanics, John Wiley and Sons, New York, Print ISBN 9780471853053, Online ISBN 9780470172766, https://doi.org/10.1002/9780470172766, 1943.
TNO: Totstandkoming GeoTOP, TNO rapport R11655, 132 pp., https://www.dinoloket.nl/sites/default/files/docs/geotop/R11655 Totstandkomingsrapport GeoTOP.pdf (last access: 31 March 2025), 2019.
Törnqvist, T. E., Wallace, D. J., Storms, J. E. A., Wallinga, J., Van Dam, R. L., Blaauw, M., Derksen, M. S., Klerks, C. J. W., Meijneken, C., and Snijders E. M. A.: Mississippi delta subsidence primarily caused by compaction of Holocene strata, Nat. Geosci., 1, 173–176, https://doi.org/10.1038/ngeo129, 2008.
van Asselen, S.: The contribution of peat compaction to total basin subsidence: implications for the provision of accommodation space in organic-rich deltas, Basin Res., 23, 239–255, https://doi.org/10.1111/j.1365-2117.2010.00482.x, 2011.
van Asselen, S., Stouthamer, E., and Van Asch, Th. W. J.: Effects of peat compaction on delta evolution: A review on processes, responses, measuring and modeling, Earth-Sci. Rev., 92, 35–51, https://doi.org/10.1016/j.earscirev.2008.11.001, 2009.
van Asselen, S., Erkens, G., and de Graaf, F.: Monitoring shallow subsidence in cultivated peatlands, Proc. IAHS, 382, 189–194, https://doi.org/10.5194/piahs-382-189-2020, 2020.
van Asselen, S., Erkens, G., Jansen, S., Fritz, C., Weideveld, S. T. J., Hessel, R., van den Akker, J. J. H., Massop, H. T. L., and Gerritsen, F.: Effects of subsurface water infiltration systems on phreatic groundwater levels in peat meadows, NOBV: Dutch National Research Programme on Greenhouse Gases in Peatlands, https://www.nobveenweiden.nl/en/findings/ (last access: 31 March 2025), 2023.
Van den Akker, J. J. H., Kuikman, P., De Vries, F., Hoving, I., Pleijter, M., Hendriks, R., Wolleswinkel, R., Simões, R., and Kwakernaak, C.: Emission of CO2 from agricultural peat soils in the Netherlands and ways to limit this emission, in: Proceedings of the 13th International Peat Congress After Wise Use – The Future of Peatlands, Vol. 1, Oral Presentations, Tullamore, Ireland, 8–13 June 2008, 645–648, https://edepot.wur.nl/159747 (last access: 1 April 2025), 2010.
Van den Born, G. J., Kragt, F., Henkens D., Rijken, B., van Bemmel B., and van der Sluis, S.: Dalende bodems, stijgende kosten, Den Haag: PBL, https://www.pbl.nl/uploads/default/downloads/pbl-2016-dalende-bodems-stijgende-kosten-1064.pdf (last access: 1 April 2025), 2016 (in Dutch).
- Abstract
- Introduction
- Methods and study site description
- Soil vertical movement measurement results
- Evaluation and discussion
- Conclusions
- Appendix A: Changes in layer thickness
- Appendix B: Additional figures for the Zegveld study site
- Data availability
- Author contributions
- Competing interests
- Disclaimer
- Acknowledgements
- Financial support
- Review statement
- References
- Abstract
- Introduction
- Methods and study site description
- Soil vertical movement measurement results
- Evaluation and discussion
- Conclusions
- Appendix A: Changes in layer thickness
- Appendix B: Additional figures for the Zegveld study site
- Data availability
- Author contributions
- Competing interests
- Disclaimer
- Acknowledgements
- Financial support
- Review statement
- References