the Creative Commons Attribution 4.0 License.
the Creative Commons Attribution 4.0 License.
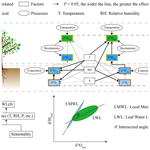
Controls on leaf water hydrogen and oxygen isotopes: a local investigation across seasons and altitude
Jinzhao Liu
Chong Jiang
Huawu Wu
Li Guo
Haiwei Zhang
Ying Zhao
The stable oxygen (δ18Oleaf) and hydrogen (δ2Hleaf) isotopes of leaf water act as a bridge that connects the hydroclimate to plant-derived organic matter. However, it remains unclear whether the source water (i.e., twig water, soil water, and precipitation) or meteorological parameters (i.e., temperature, relative humidity, and precipitation) are the dominant controls on δ18Oleaf and δ2Hleaf. Here, we reported a seasonal analysis of δ18Oleaf and δ2Hleaf together with isotopes from potential source waters and meteorological parameters along an elevation transect on the Chinese Loess Plateau. We found that δ2Hleaf values were more closely correlated with source water isotopes than δ18Oleaf values, whereas δ18Oleaf and δ2Hleaf values were similarly correlated with meteorological parameters along the elevation transect. Dual-isotope analysis showed that the δ18Oleaf and δ2Hleaf values were closely associated because of their similar altitudinal and seasonal responses, generating a well-defined isotope line relative to the local meteoric water line (LMWL). We also compared the measured δ18Oleaf and δ2Hleaf values with values predicted by the Craig–Gordon model and found no significant differences between them. We demonstrate that the first-order control on δ18Oleaf and δ2Hleaf values was the source water, and the second-order control was the enrichment associated with biochemical and environmental factors on the Loess Plateau.
- Article
(4284 KB) - Full-text XML
-
Supplement
(823 KB) - BibTeX
- EndNote
The stable isotope compositions of oxygen and hydrogen (δ18O and δ2H, respectively) are increasingly being used as powerful tracers to follow the path of water from its input as precipitation, movement through the soil, and ultimately to its release as soil evaporation and leaf transpiration (Penna and Van Meerveld, 2019). Leaf water transpiration plays a key role in regulating the water balance at scales ranging from catchment to global. Terrestrial plants can enrich heavier isotopes (2H and 18O) in leaf water via evaporative fractionation through the stoma (Helliker and Ehleringer, 2000; Liu et al., 2015; Cernusak et al., 2016), which is highly dependent on atmospheric conditions (e.g., temperature and relative humidity) and biophysiological processes (Farquhar et al., 2007; Kahmen et al., 2011; Cernusak et al., 2016). Subsequently, the isotopic signals from leaf water are integrated into plant organic matter, such as cellulose (e.g., Barbour, 2007; Lehman et al., 2017) and leaf wax (Liu et al., 2016, 2021b), as powerful proxies used for paleoclimate reconstruction (Pagani et al., 2006; Schefuß et al., 2011; Hepp et al., 2020). However, although leaf water isotopes are the fundamental parameters in ecohydrology and organic biosynthesis, an adequate understanding of controls of leaf water isotopes and the role of source water and hydroclimate in determining leaf water isotopes is still lacking.
δ18Oleaf and δ2Hleaf values are influenced first by a plant's source water (mainly water taken up by roots from the soil; Cernusak et al., 2016; Barbour et al., 2017; Munksgaard et al., 2017; Liu et al., 2022) and second by the enrichment associated with transpiration (Munksgaard et al., 2017). Soil water for terrestrial plants generally originates from local precipitation, and precipitation isotopes vary spatially and temporally, being subject to controls including temperature, altitude, latitude, distance from the coast, and amount of precipitation (Bowen, 2010; Bowen and Good, 2015; Cernusak et al., 2016). More specifically, soil water isotopes are determined by a mixture of individual precipitation events with distinct isotopic signals and are also affected by evaporation, both of which lead to the development of isotopic gradients in soil water with depth (Allison et al., 1983; Liu et al., 2015). Many studies have shown that the δ18O and δ2H values of root/xylem water can be used to characterize the water sources used by plants (Rothfuss and Javaux, 2017; Wu et al., 2018; Wang et al., 2019; Amin et al., 2020; Zhao et al., 2020; Liu et al., 2021a). These studies rested substantially on the assumption that no isotopic fractionation of δ18O and δ2H values occurs during water uptake by plant roots (Dawson and Ehleringer, 1991; Ehleringer and Dawson, 1992; Chen et al., 2020), except in saline or xeric environments (Lin and Sternberg, 1993; Ellsworth and Williams, 2007). Some recent studies showed, however, that the occurrence of isotopic fractionation during root water uptake was probably more common than previously thought, especially with respect to δ2H values (Zhao et al., 2016; Wang et al., 2017; Barbeta et al., 2019; Poca et al., 2019; Liu et al., 2021a, 2022).
In addition to plant source water, leaf water is also isotopically enriched through the evaporative process during transpiration. The enrichment of 18O and 2H by leaf water transpiration can be predicted using the Craig–Gordon model (C–G model). This model was initially proposed to describe the evaporative enrichment of a freely evaporating water body (Craig and Gordon, 1965) and has been modified for plant leaves under steady-state conditions (Dongmann et al., 1974; Farquhar and Cernusak, 2005). However, the C–G model fails to explain the intra-leaf heterogeneity of δ18Oleaf and δ2Hleaf (Cernusak et al., 2016; Liu et al., 2021b), which is currently described using a two-pool model (Leaney et al., 1985; Song et al., 2015) and/or an advection–diffusion model, as the Péclet effect (Farquhar and Lloyd, 1993; Farquhar and Gan, 2003). Subsequently, more complicated models have been developed to cover non-steady-state conditions (Ogée et al., 2007). These models emphasize a mechanistic understanding of leaf water isotopic fractionation, but the relevant parameters cannot be strictly constrained or precisely monitored, which hinders the use of these models under natural conditions (Plavcová et al., 2018).
This study combined the effects of measured source water isotopes and C–G model-predicted transpiration on δ18Oleaf and δ2Hleaf values. Our objectives were to deepen the understanding of the controls on the δ18Oleaf and δ2Hleaf across different seasons. Based upon these objectives, we repeatedly sampled soils, twigs, and leaves in May, July, and September (representing spring, summer, and fall, respectively) from the same 10 plots distributed along an elevation transect. Simultaneously, we obtained the relevant meteorological parameters (e.g., temperature, relative humidity, and precipitation) from sites close to the sampling plots along the transect and used these to predict the δ18Oleaf and δ2Hleaf values. The combined analysis of concurrent measurements of δ18O and δ2H values in soil water, twig water, and leaf water with the predicted δ18O and δ2H values of leaf water from the C–G model associated with the surrounding meteorological parameters will help to identify the factors that control δ18Oleaf and δ2Hleaf values. Furthermore, we performed an isotope-based line analysis of the dual δ18O and δ2H values of leaf water, associated with altitude and seasonality. This study will improve our understanding of the environmental signals preserved within the δ18O and δ2H values extracted from plant organic biomarkers associated with leaf water.
2.1 Study area
The Qinling Mountains form the dividing line between northern and southern China and mark the boundary between the watersheds of the Yellow and Yangtze rivers. Mt. Taibai (Fig. 1; 33.96∘ N, 107.77∘ E) rises to 3767 m a.s.l. (above sea level) and is the peak in the Qinling Mountains; it has a warm temperate ecosystem characterized by a rich diversity of flora and fauna. The mean annual temperature at the bottom of Mt. Taibai is 12.9 ∘C, and the mean annual precipitation is 609.5 mm (Zhang and Liu, 2010). The climate, soil, and vegetation vary significantly along our slope transect, exhibiting a remarkable vertical geo-ecological zonation (Fig. 1). The area contains a variety of climate zones: warm temperate (<1300 m a.s.l.), temperate (1300–2600 m a.s.l.), cool temperate (2600–3350 m a.s.l.), and alpine (>3350 m a.s.l.). The soil types vary from yellow loess soil at low elevations, spectacular rocky outcrops at middle elevations, and glacial remnants at high elevations. Vegetation along the transect is mainly coniferous and broadleaf forests, as well as alpine and subalpine vegetation (Fig. 1; Liu, 2021). The dominant species range from Quercus variabilis, Q. aliena, Betula albosinensis, B. utilis, Abies fargessi, and Larix chinensis forests to Rhododendron clementinae and R. concinnum alpines (Fig. S1 in the Supplement).
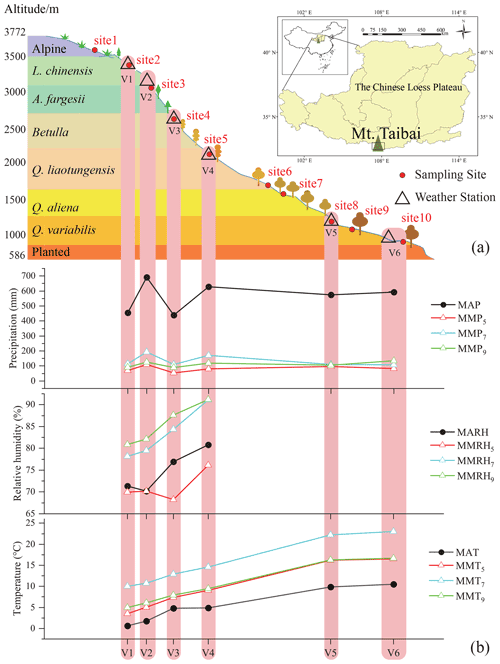
Figure 1Sample sites (red dots) and weather stations (open triangles) that are distributed along vertical vegetation zones across the Mt. Taibai transect on the Chinese Loess Plateau (a). The meteorological parameters (precipitation, temperature, and relative humidity) vary with stations along the elevation transect (b). Mean annual (MAP, MAT, MARH) and monthly (MMP, MMT, MMRH) precipitation, temperature, and relative humidity. The subscripts refer to the month. The vertical vegetation distribution was adopted from Liu (2021).
2.2 Sampling strategy
Plants and soils were sampled in May, July, and September 2020, and samples were collected from 10 plots (3×3 m) covering all the vegetation zones along the northern slope of Mt. Taibai, extending from 608 to 3533 m a.s.l. (Fig. 1). Among the plots, six sites (i.e., sites 2, 3, 4, 5, 8, and 10; Fig. 1) were selected as being the closest to the weather stations along the elevation transect, and they were used in order to obtain the in situ meteorological data for analysis. For the plants, one or two dominant deciduous and coniferous trees were chosen in each plot across the vegetation zone (Fig. S1). Several large leaves and suberized twigs were collected for each species. Between 3 to 10 large leaves were chosen for sampling, and a small number was collected in broadleaf forests and a large number in coniferous forests, depending on leaf size. The leaf samples were conducted in the context of the intact leaves because of the likely isotopic gradients within a leaf (Helliker and Ehleringer, 2000; Liu et al., 2016). Our sampling period was between 12:00 and 15:00 CST, because maximum diurnal enrichment of the leaf water isotopic composition occurs during this part of the day (Romero and Feakins, 2011; Liu et al., 2021). The twigs were collected simultaneously by cutting suberized twigs, and all of the twigs were cut into samples that were 3–4 cm long. The leaf and twig samples were immediately placed into glass vials with screw caps and sealed with polyethylene parafilm. For the soils, three surface soil samples (less than 10 cm deep) were collected from around the sampled plants using a small metal scoop at each plot. All sampling plots were located on slopes far from rivers and surface water bodies, which ensured that the soil water in each plot was derived exclusively from precipitation. Although the surface soil layers were collected only as the representatives of soil water in this study, these samples could provide a relatively good source of water for the plants, as supported by a prior study conducted along the same elevation transect (Zhang and Liu, 2010). The soil samples were tightly sealed in a polyethylene zipper bag on-site. All plant and soil samples were stored in a cool box (∼4 ∘C) in the field and immediately transported to the laboratory. The altitude of each plot was determined using a handheld GPS unit with an error of ±5 m.
2.3 Isotope analysis
The water in the plant and soil samples was extracted using an automatic cryogenic vacuum extraction system (LI-2100 Pro, LICA United Technology Limited, Beijing, China). The auto-extraction process was set for 3 h, and the extraction rate of water from the samples was more than 98 %. The isotopic composition of the soil water was measured using a Picarro L2130-i isotope water analyzer (Sunnyvale, CA, USA) at the State Key Laboratory of Loess and Quaternary Geology, Institute of Earth Environment, Chinese Academy of Sciences. The analytical accuracies were ±0.1 ‰ for δ18O and ±1 ‰ for δ2H. An isotope ratio mass spectrometer was coupled to a high-temperature conversion elemental analyzer (HT2000 EA-IRMS, Delta V Advantage; Thermo Fisher Scientific, Inc. USA) to take isotopic measurements of twig and leaf water at the Huake Precision Stable Isotope Laboratory on the campus of Tsinghua Shenzhen International Graduate School. The measurement precisions were ±0.2 ‰ and ±1 ‰ for δ18O and δ2H, respectively. The isotopic composition of δ18O and δ2H is expressed as an isotopic ratio:
where δsample represents δ18O or δ2H, and Rsample and Rstandard indicate the ratio of or of the sample and standard, respectively. The δ18O and δ2H values are reported relative to the Vienna Standard Mean Ocean Water (VSMOW). In addition, the mean monthly δ18O and δ2H values of precipitation were determined using the Online Isotopes in Precipitation Calculator (Bowen and Revenaugh, 2003).
2.4 Modeling isotopes of leaf water
The C–G equation can be approximated as follows (Cernusak et al., 2022):
where δe is the predicted δ18O and δ2H values at the evaporative sites within leaves, δs is the δ18O and δ2H values of source water (equivalent to twig water in our study), ε+ is the equilibrium fractionation between liquid water and vapor, and εk is the kinetic fractionation during the diffusion of vapor through the stomata and the boundary layer.
In our analysis, we calculated Δv (the enrichment of atmospheric vapor relative to source water) as , and the values of Δv are often close to at the isotopic steady state (Barbour, 2007; Cernusak et al., 2016); therefore, we can calculate δv as . In addition, is the ratio of the water vapor pressure fraction in the air relative to that in the intercellular spaces and is equal to the relative humidity (RH) in the air at steady state (Cernusak et al., 2022). Thus, Eq. (2) can be derived as
where δs represents the isotopic values of twig water, and h is the mean annual or monthly RH (MARH or MMRH) in this study. The equilibrium fractionation (ε+) varies as a function of temperature (Bottinga and Craig, 1969) and can be equated to δ18O and δ2H, as follows (Majoube, 1971):
The kinetic fractionation (εk) can be calculated for δ18O and δ2H as (Farquhar et al., 2007; Cernusak et al., 2016)
where rs and rb are the resistances of the stomatal and boundary layers, respectively (i.e., the inverse of the conductance of the stomatal and boundary layers). Previous studies have found stomatal and boundary layer conductance values of 0.49 and 2.85 mol m−2 s−1, respectively (Cernusak et al., 2016; Munksgaard et al., 2017), resulting in and values of 26.7 and 23.8, respectively.
2.5 Statistical analysis
Statistical analysis (i.e., the mean, maximum, and minimum values, as well as the standard deviation) of the isotopes extracted from the precipitation, soil, twig, and leaf samples was performed to define the range and distribution of the δ18O and δ2H values across the seasons. The Pearson correlation method was used to assess the correlations between the δ18O and δ2H values among the different water types (i.e., precipitation, soil water, twig water, and leaf water). Hierarchical cluster analysis was used to show the relationships among δ18Oleaf and δ2Hleaf values and potential source water isotopes (δ18O and δ2H values in precipitation, soil water, twig water, and leaf water), and meteorological parameters such as mean annual and monthly precipitation (MAP and MMP), mean annual and monthly temperature (MAT and MMT), and mean annual and monthly relative humidity (MARH and MMRH). A one-way analysis of variance (ANOVA) combined with a post hoc Tukey's least significant difference (LSD) test were performed to identify the significant differences in the isotopic compositions of precipitation, soil, twig, and leaf waters across the months. Comparisons of the relationships of δ18O and δ2H in the soil and leaf water were performed using covariance analysis (ANCOVA) to compare slopes across months. The structural equation model (SEM) was used to explain the respective effects of source waters (i.e., twig water, soil water, and precipitation) and meteorological parameters (i.e., temperature, precipitation, and RH) on δ18Oleaf and δ2Hleaf values. The validated SEMs generated a good model fit, as indicated by a non-significant χ2 test (p>0.05), a high comparative fit index (CFI > 0.95), and a low root mean square error of approximation (RMSEA < 0.05). A special SEM was constructed based on the Mantel R values in AMOS (version 24.0.0). Moreover, we used the Hybrid Single-Particle Lagrangian Integrated Trajectory (HYSPLIT) model (Draxler and Rolph, 2003) to calculate air mass back trajectory for the central site (34.13∘ N, 107.83∘ E, 2270 m a.s.l.) in the study area. These trajectories were initiated four times daily (at 00:00, 06:00, 12:00, and 18:00 CST), and their air parcel was released at 2300 m a.s.l. for May, July, and September 2020 and moved backwards by winds for 120 h (5 d).
3.1 Differing response of δ18O and δ2H values of leaf water
The measured δ18O and δ2H values of leaf water responded differently to source water isotopes (Fig. 2a) and meteorological parameters (Fig. 2b) across the seasons. The leaf water δ18O and δ2H values (δ18Oleaf and δ2Hleaf) were clustered with those of the twig water (δ18Otwig and δ2Htwig; Fig. 2a) and with MARH, MAT, and MMT (Fig. 2b). The δ2Hleaf values were more closely correlated with isotopes of the potential source waters (e.g., twig water, soil water, and precipitation) than the δ18Oleaf values in different months (Fig. 2a). In contrast, leaf water δ18O and δ2H values were correlated with meteorological parameters (Fig. 2b) throughout the study period. These correlations were more significant in summer (July) and autumn (September) than in spring (May).
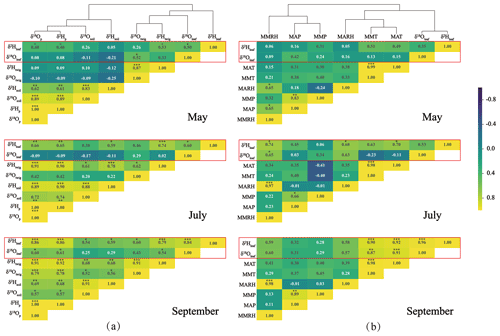
Figure 2Heat maps of correlations (r) between leaf water δ18O and δ2H values and potential source water δ18O and δ2H values (twig water, soil water, and precipitation δ18O and δ2H values; a), and meteorological parameters (e.g., MAP, MMP, MAT, MMT, MARH, MMRH). The hierarchical cluster analysis of the isotopes of leaf water and source water (a) and meteorological parameters (b). The subscripts (p, soil, twig, leaf) refer to precipitation, soil water, twig water, and leaf water. * Corrected significance at p<0.05, corrected significance at p<0.01, and corrected significance at p<0.001.
3.2 Comparisons of measured and predicted δ18O and δ2H values of leaf water
The δ18Oleaf and δ2Hleaf values predicted by the C–G model were compared with the measured δ18O and δ2H values across all three months (Fig. 3). The C–G model explained 49 % and 70 % of the observed variations in the δ18Oleaf and δ2Hleaf values, respectively (Fig. 3a and c). The slopes of the relationships for both δ18O and δ2H values of leaf water were less than one, which suggests that part of the bulk leaf water is derived from unenriched vein water. However, there were no significant differences in δ18Oleaf (p=0.54; Fig. 3b) and δ2Hleaf values (p=0.93; Fig. 3d) between the C–G model predicted values and the measured values.
3.3 Variations of δ18O and δ2H values of different waters with seasons and altitude
There was a significant correlation between δ18Oleaf and δ2Hleaf values (R2=0.81, p<0.01; Fig. 4), with significant clusters of δ18Oleaf and δ2Hleaf values across the months, and values were higher in May, intermediate in July, and lower in September (Fig. 4). Within each month, the δ18Oleaf and δ2Hleaf values were depleted in 2H and 18O at higher altitudes relative to lower altitudes. Likewise, the potential types of source water (i.e., twig water, soil water, and precipitation) exhibited consistent variations across the months, showing values that were relatively higher in May, intermediate in July, and lower in September (Fig. S1). The correlations between δ18O and δ2H values among the source waters were also significant (Fig. S2). Nevertheless, the slopes and coefficients of determination (R2) between the δ18O and δ2H values showed a decrease for precipitation, soil water, twig water, and leaf water from the three sampling months, except for soil water in May (Fig. S2). In addition, the ANCOVA showed no significant differences for the regression lines for precipitation (df=0.47, F=2.49, ), twig water (df=53.2, F=0.42, ), and leaf water (df=437.3, F=2.78, ) across the study months but a significant difference for soil water across the months (df=308.8, F=10.9, p<0.05).
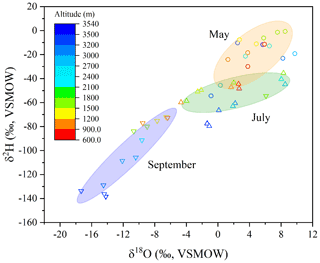
Figure 4Correlation of leaf water δ18O and δ2H values across months and altitude. Leaf water δ18O and δ2H values were higher in May, intermediate in July, and lower in September, and while within each month, those isotopic values were relatively lower at high altitudes and higher in lower altitudes.
4.1 δ18O and δ2H values of leaf water
A recent global meta-analysis indicated that δ18Oleaf and δ2Hleaf values reflect environmental drivers differently and showed that δ2Hleaf values more strongly reflect xylem water and atmospheric vapor δ2H values, whereas δ18Oleaf values more strongly reflect air relative humidity (Cernusak et al., 2022). Seasonal and localized observations along an elevation transect on the Chinese Loess Plateau supported these different responses of δ18Oleaf and δ2Hleaf to the isotopic composition of the source water and meteorological conditions (Fig. 2). This is likely due to the fact that variation in precipitation isotopic values compared with that in leaf water evaporative enrichment is larger for δ2Hleaf than δ18Oleaf (Cernusak et al., 2022). In addition, we found stronger correlations between δ2Hleaf and isotope values of the source water (twig water, soil water, and precipitation) than between δ18Oleaf values and the source water isotope values (Fig. 2a). This is consistent with the global meta-analysis results (Cernusak et al., 2022). However, our localized study did not show a significantly different response of δ18Oleaf and δ2Hleaf values to meteorological parameters, which responded at almost equivalent magnitudes (Fig. 2b). These observations suggest that plant organic isotopic proxies such as leaf wax (Sachse et al., 2012; Liu et al., 2016) and cellulose (Barbour, 2007; Lehman et al., 2017), which originate from δ18Oleaf and δ2Hleaf values, can provide comparative information that indicates climatic signals (e.g., temperature, RH, and precipitation) in natural archives. These results argued with the recent global meta-analysis that δ18Oleaf and δ2Hleaf values reflect climatic parameters (i.e., RH and temperature) differently (Cernusak et al., 2022). The stronger correlations for δ2Hleaf values than δ18Oleaf values with isotopic values of the source water were likely, because the δ2Hleaf values are ultimately determined only by precipitation δ2H (Sachse et al., 2012; Liu et al., 2016), whereas the δ18Oleaf values are affected by a mixture of precipitation δ18O and atmospheric factors (O2 and CO2) (Barbour, 2007; Cernusak et al., 2016). However, the comparative responses of both δ2Hleaf and δ18Oleaf values to climatic parameters were probably due to the same conditions surrounding the leaf.
The results of the cluster analysis showed that the isotope values of leaf water (δ18Oleaf and δ2Hleaf) and twig water (δ18Otwig and δ2Htwig) were clustered into one group, but those of soil water (δ18Osoil and δ2Hsoil) and precipitation (δ18Op and δ2Hp) were clustered into another (Fig. 2a). This indicates that the direct source water of δ18Oleaf and δ2Hleaf should be δ18Otwig and δ2Htwig, providing the source water isotope basis for the C–G model. In the C–G model (see Eq. 2), besides the source water isotopes, the equilibrium fractionation factor (ε+) and atmospheric vapor enrichment (Δv) depend on the temperature at the isotopic steady state. Thus, the δ18Oleaf and δ2Hleaf values were predicted to be associated primarily with temperature, RH, and source water, which is consistent with the results from the cluster analysis that the δ18Oleaf and δ2Hleaf values were clustered with temperature (MAT and MMT) and RH (MARH; Fig. 2b). Based on the C–G model, we plotted the measured and predicted δ18Oleaf and δ2Hleaf values (Fig. 3a and c) and observed no significant differences between them (Fig. 3b and d). This is because our three-repeated samplings occur during the day when leaf water is generally near an isotopic steady state when chloroplasts are mostly located near the evaporative sites (Cernusak et al., 2016). The non-steady-state effects on leaf water isotopes were expected at night because of low stomatal conductance (Cernusak et al., 2005, 2016; Cuntz et al., 2007). Although the slopes of the predicted and measured δ18Oleaf and δ2Hleaf values were less than one, the C–G model still provides a reasonable framework for guiding the analysis of the different controls on δ18Oleaf and δ2Hleaf values.
4.2 Dual δ18O and δ2H plots of leaf water
There was a significant linear correlation between the δ18Oleaf and δ2Hleaf values, with remarkable clusters associated with the three months studied (Fig. 4). As is well known, the local meteoric water line (LMWL), generated by precipitation δ18O and δ2H values at the local scale, serves as an important reference line for intercomparisons of different waters. Furthermore, the regression lines of the δ18O and δ2H values from soil water, twig water, and leaf water (Fig. S2) suggest that the leaf water isotopes could well inherit isotopic signals of source waters that originate from twig water, soil water, and ultimately precipitation. The slopes and intercepts of the δ18O and δ2H values decreased significantly from precipitation, soil water, twig water, and leaf water for each month, except for soil water in May (Fig. S2). Such patterns have been observed in many previous calibration studies (Brooks et al., 2010; Evaristo et al., 2015; Sprenger et al., 2016, 2017; Wang et al., 2017; Benettin et al., 2018; Barbeta et al., 2019; Penna and Van Meerveld, 2019; Liu et al., 2021a, 2022). The slopes of the LMWLs were lower in July (6.79) than in May (7.04) and September (6.85) but were not significantly different (ANCOVA test: df=0.47, F=2.49, ). This suggests that the local water vapor from precipitation was derived from the same source across the seasons but was subject to different intensities of evaporation as the temperature changed throughout the seasons (Li et al., 2019; Wu et al., 2019, 2021). The slopes of the δ18O and δ2H values from the soil, twig, and leaf waters were much smaller than the LMWLs across the study months due to the secondary evaporation in the other water types.
In the dual isotope plot of leaf water, there were well-defined clusters of δ18Oleaf and δ2Hleaf values across the three months: 18O and 2H were depleted in September, there were intermediate values in July, and 18O and 2H were enriched in May (Fig. 4). When focusing on each month, relatively higher isotopic values occurred at low elevations, but lower isotopic values were present at high elevations despite there being no, or only weak, correlations between the δ18Oleaf and δ2Hleaf values and altitude (Fig. S3). The correlations between the δ18Oleaf and δ2Hleaf values and altitude, and between the δ18Otwig and δ2Htwig values and altitude, were not significant across the three months; however, the δ18Op and δ2Hp, and also the δ18Osoil and δ2Hsoil values, were significantly correlated with altitude (Fig. S3), indicating that besides source water (precipitation and soil water), other factors associated with plants also affect δ18Oleaf and δ2Hleaf values.
The dual isotope plot of δ18Oleaf and δ2Hleaf values shows a significant isotope line, (R2=0.81, p<0.01; Fig. 4), but shallower slopes (3.53, 1.86, and 2.81 in May, July, and September, respectively) of δ18Oleaf and δ2Hleaf values were observed across the seasons (Fig. S2). Such a correlation was supported by a recent study that conducted consecutive measurements of δ18O and δ2H values in xylem and leaf water in Switzerland and indicated that leaf water provided great potential to determine the source water of plants (Benettin et al., 2021). Our study showed remarkable clusters in the measured (Fig. 4) and the C–G model-predicted (Fig. 3) δ18Oleaf and δ2Hleaf values across the months and the consistencies of respective δ18Oleaf and δ2Hleaf values with potential source water isotopes across months (Fig. S1). These findings of temporally consistent dynamics among the water types (i.e., precipitation, soil water, twig/stem water, and leaf water) have been observed in a number of previous studies (Phillips and Ehleringer, 1995; Cernusak et al., 2005; Sprenger et al., 2016; Berry et al., 2017; Liu et al., 2021a).
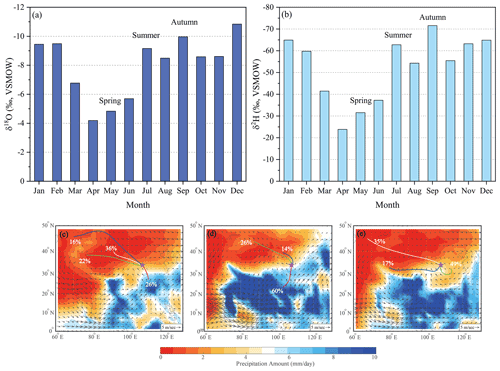
Figure 5Variation of monthly mean precipitation δ18O (a) and δ2H (b) values at Xi'an station from the Global Network of Isotopes in Precipitation (GNIP) and cluster mean of moisture transport routes using the HYSPLIT model in May (c), July (d) and September (e), 2020. The background in (c)–(e) is the average precipitation (mm d−1) and 850 hPa wind vectors (arrows, m s−1) in May (c), July (d), and September (e) in AD 1979–2016 based on the database of the Global Precipitation Climatology Center (GPCC) (Becker et al., 2011) and the Modern-Era Retrospective analysis for Research and Applications (Rienecker et al., 2011).
The isotopic inheritance from precipitation to leaf water indicates that seasonal variations of δ18Op and δ2Hp values are the first-order control on the temporal patterns observed in leaf water. The seasonal dynamics of the δ18Op and δ2Hp values reflect the combined effects of factors such as temperature, altitude, and precipitation amount, which are associated with orographic conditions, as well as sub-cloud evaporation, moisture recycling, and differences in the vapor source (Dansgaard, 1964; McGuire and McDonnell, 2007; Li et al., 2016; Penna and Van Meerveld, 2019; Wu et al., 2019). In this study, we used the HYSPLIT model to demonstrate the ultimate cause of the seasonal variations of δ18Oleaf and δ2Hleaf values, that is, the monthly dynamics of the δ18Op and δ2Hp values. The monthly variations of the δ18Op and δ2Hp values from the Global Network for Isotopes in Precipitation (GNIP, http://www.iaea.org/, last access: 18 April 2021) at Xi'an station (AD 1985–1992), which is ∼100 km from our study transect, were enriched in 18O and 2H in May relative to July and September (Fig. 5a and b). The cluster mean of the moisture transport routes from HYSPLIT (Draxler and Rolph, 2003) and the climatological 850 hPa wind vectors showed that the primary moisture sources were from western China and central Asia in May, the China–India Peninsula and the Bay of Bengal, and local moisture recycling and convection (Fig. 5c–e). The seasonal variations in δ18Op and δ2Hp values are consistently related to the onset, advancement, and retreat of the Asian summer monsoon and associated changes in the large-scale monsoon circulation (e.g., Zhang et al., 2020, 2021). As the summer monsoon starts in mid-May, the rainfall season starts in southern China; however, our study area is controlled mainly by moisture from the westerlies (Chiang et al., 2015) with relatively higher vapor, δ18Op, and δ2Hp values (Fig. 5a–c). In July, the summer monsoon reaches its strongest phase, and the rainfall belt shifts to central and northern China, where the southerly wind brings plenty of moisture from the China–India Peninsula and the Bay of Bengal with lower vapor, δ18Op, and δ2Hp values (Fig. 5a, b, and d). When the summer monsoon withdraws in September, the study area is controlled mainly by local moisture recycling and convection (Fig. 5e). Soil water, stored after the June–August monsoon rainfall with its lower δ18O and δ2H values, results in even lower δ18Op and δ2Hp values in September than in July (Fig. S1), causing significantly lower δ18O and δ2H values of leaf water (Fig. 4).
4.3 Framework of controls for δ18O and δ2H values of leaf water
To delineate the mechanisms that control the δ18Oleaf and δ2Hleaf values, we used the SEMs to quantify the complex interactions among δ18Oleaf or δ2Hleaf values, source waters, and meteorological parameters (Fig. 6). The coefficients of determination (R2) were 0.48 and 0.71 for the δ18Oleaf and δ2Hleaf values, respectively, indicating that the models explained more variance for δ2Hleaf values than δ18Oleaf values (Fig. 6). The SEMs showed that potential source waters (i.e., twig water, soil water, and precipitation) had stronger effects on δ2Hleaf relative to δ18Oleaf values, while the meteorological parameters showed weak effects on both δ18Oleaf and δ2Hleaf values (a little larger for δ2Hleaf than δ18Oleaf values). This is consistent with our above correlation analysis (Fig. 2). Surprisingly, the MMT had significant effects on δ18Op and δ2Hp values, suggesting that temperature plays a key role in determining δ18Op and δ2Hp values, but this finding is not discussed further here. Collectively, the SEMs also showed that source water exerts the first-order control but affects δ18Oleaf and δ2Hleaf differently; the meteorological parameters had a weak control on δ18Oleaf and δ2Hleaf, with a more substantial effect on δ2Hleaf than δ18Oleaf values.
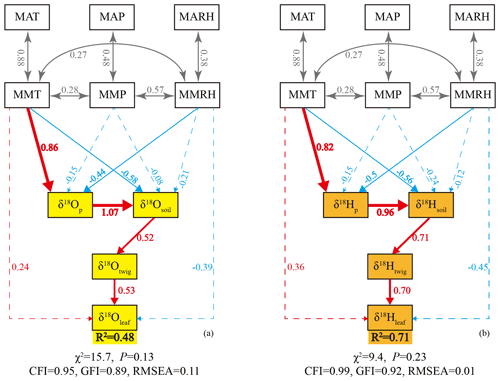
Figure 6Structural equation model (SEM) of leaf water δ18O (a) and δ2H (b) values. The structural equation models considered all plausible pathways. Solid lines indicate significant positive (red) or negative (blue) effects, and dashed lines indicate non-significant effects. Grey lines indicate correlations between two variables. Numbers on the arrow indicate significant standardized path coefficients, proportional to the arrow width. The coefficients of determination (R2) represent the proportion of variance explained by the model.
A schematic representation of the controls on δ18Oleaf and δ2Hleaf values (respective and dual) is shown in Fig. 7. It involves multiple processes associated with the hydroclimatic and biochemical factors that affect δ18Oleaf and δ2Hleaf values. The meteorological parameters (temperature, RH, and precipitation) exerted distinct effects on the δ18O and δ2H values of the source water and, thus, on the δ18Oleaf and δ2Hleaf values, as demonstrated above by the SEM. Significant isotopic fractionation occurred mainly at two key locations across the vertical soil profiles and leaf architectures from precipitation to leaf water. First, an isotopic gradient across the vertical soil profile appeared because of evaporation from the surface soil layers (Ehleringer and Dawson, 1992; Goldsmith et al., 2012; Evaristo et al., 2015). This evaporative isotopic fractionation causes a linearly isotopic trajectory down the soil profile (Goldsmith et al., 2012; Rothfuss and Javaux, 2017; Wu et al., 2018; Wang et al., 2019; Amin et al., 2020; Zhao et al., 2020; Liu et al., 2021a). Second, there were significant isotopic heterogeneities because of transpiration associated with the δ18Oleaf (Helliker and Ehleringer, 2000; Farquhar and Gan, 2003; Gan et al., 2003; Song et al., 2015) and δ2Hleaf values (Šantrůček et al., 2007; Liu et al., 2016, 2021b) within a leaf, which depends substantially on veinal structures (Liu et al., 2021b). The within-leaf heterogeneity of the δ18Oleaf and δ2Hleaf values can be explained using the Péclet-modified C–G model (Gan et al., 2003; Farquhar and Gan, 2003; Cernusak et al., 2005, 2016). Collectively, the soil evaporation and leaf transpiration produce isotopic enrichment above source water (precipitation or soil water). Soil evaporation leads to an isotopic gradient across the vertical soil profile, providing water sources for plant root uptake without isotope fractionation during the process (Dawson and Ehleringer, 1991; Ehleringer and Dawson, 1992; Chen et al., 2020). During water transport between roots and leaf petioles, isotopic compositions of xylem water remain unaltered from those in soils (i.e., soil immobile water), until it reaches the leaf, which undergoes water loss (Ehleringer and Dawson, 1992). Within the leaf, transpiration leads to significant isotopic enrichment (Helliker and Ehleinger, 2000; Liu et al., 2015; Cernusak et al., 2016), which is highly dependent on meteorological parameters (e.g., temperature and relative humidity). However, the meteorological parameters varied with altitude and seasonality, yielding an isotopic leaf water line (LWL) in the dual-isotope plot (Fig. 4). The LWL provides an important baseline for leaf-derived organic matter such as cellulose (e.g., Barbour, 2007; Lehman et al., 2017) and leaf wax (Liu et al., 2016, 2021). Overall, the LWL is controlled primarily by altitude and seasonality, as these are the main influencers of the hydroclimatic factors.
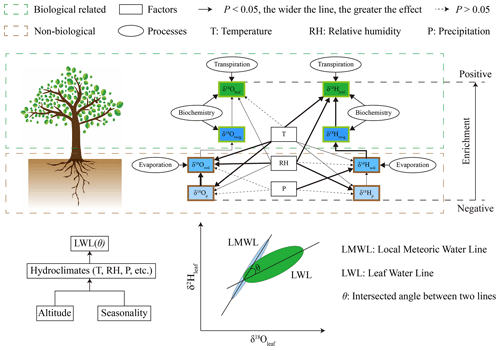
Figure 7Schematics of the respective and dual isotopes of δ18O and δ2H values from precipitation to leaf water, associated with physical (evaporation at soil profile and transpiration at leaf level) and biochemical processes. The dual isotopes of δ18O and δ2H values yield an isotopic water line, the slope of which was lower than the LMWL. The intersected angle varied with hydroclimates, associated with altitude and seasonality.
Along an elevation transect on the Chinese Loess Plateau, precipitation, soil water, twig water, and leaf water were repeatedly sampled to explore the controls on δ18Oleaf and δ2Hleaf values associated with meteorological parameters and source water. The effects of meteorological parameters and source water on δ18Oleaf and δ2Hleaf values were different, and the dual δ18Oleaf and δ2Hleaf plot generated an isotopic line. We found that δ2Hleaf values were more closely correlated with source water isotopes than δ18Oleaf values, whereas δ18Oleaf and δ2Hleaf values were similarly correlated with meteorological parameters along the elevation transect. The observations suggest that plant organic isotopic proxies such as leaf wax and cellulose originating from δ18Oleaf and δ2Hleaf values can provide comparative climatic information on the Loess Plateau of China. Additionally, the dual-isotope analysis showed that the δ18Oleaf and δ2Hleaf values were closely correlated because of their similar altitudinal and seasonal responses. The first-order control on δ18Oleaf and δ2Hleaf values was the source water (i.e., precipitation), and the meteorological parameters had a comparable effect on both δ18Oleaf and δ2Hleaf values, which varied with altitude and season across the transect on the Loess Plateau. In the future, we will investigate the relationships of intersection angle θ with hydroclimatic and biochemical factors.
The data can be made available by contacting the corresponding author.
The supplement related to this article is available online at: https://doi.org/10.5194/hess-27-599-2023-supplement.
JL conceived the idea of the research and performed the data analysis. JL, HW, and HZ wrote the manuscript. LG and YZ edited the paper. JL and CJ performed the lab work. All authors contributed to discuss the results.
The contact author has declared that none of the authors has any competing interests.
Publisher's note: Copernicus Publications remains neutral with regard to jurisdictional claims in published maps and institutional affiliations.
We thank Xijing Cao and Meng Xing for help with laboratory assistance and Yao Cheng for the help in the field. We thank Jeffrey J. McDonnell and Lucas A. Cernusak for discussing and editing the paper. We also thank the Shaanxi Meteorological Bureau for supporting the meteorological data along an elevation transect.
This research has been supported by the National Natural Science Foundation of China (grant no. 42073017) and the Chinese Academy of Sciences (grant nos. XDB40000000, XAB2019B02, and ZDBS-LY-DQC033).
This paper was edited by Lixin Wang and reviewed by two anonymous referees.
Allison, G., Barnes, C., and Hughes, M.: The distribution of deuterium and 18O in dry soils 2. Experimental, J. Hydrol., 64, 377–397, 1983.
Amin, A., Zuecco, G., Geris, J., Schwendenmann, L., McDonnell, J. J., Borga, M., and Penna, D.: Depth distribution of soil water sourced by plants at the global scale: a new direct inference approach, Ecohydrology, 13, e2177, https://doi.org/10.1002/eco.2177, 2020.
Barbeta, A., Jones, S. P., Clavé, L., Wingate, L., Gimeno, T. E., Fréjaville, B., Wohl, S., and Ogée, J.: Unexplained hydrogen isotope offsets complicate the identification and quantification of tree water sources in a riparian forest, Hydrol. Earth Syst. Sci., 23, 2129–2146, https://doi.org/10.5194/hess-23-2129-2019, 2019.
Barbour, M. M.: Stable oxygen isotope composition of plant tissue: a review, Funct. Plant Biol., 34, 83–94, 2007.
Barbour, M. M., Farquhar, G. D., and Buckley, T. N.: Leaf water stable isotopes and water transport outside the xylem, Plant Cell Environ., 40, 914–920, 2017.
Becker, A., Finger, P., Meyer-Christoffer, A., Rudolf, B., and Ziese, M.: GPCC Full Data Reanalysis Version 6.0 at 1.0: Monthly Land-Surface Precipitation from RainGauges Built on GTS-Based and Historic Data, GPCC – Global Precipitation Climatology Centre, Berlin, Germany, https://psl.noaa.gov/data/gridded/data.gpcc.html (last access: 5 March 2021), 2011.
Benettin, P., Volkmann, T. H. M., von Freyberg, J., Frentress, J., Penna, D., Dawson, T. E., and Kirchner, J. W.: Effects of climatic seasonality on the isotopic composition of evaporating soil waters, Hydrol. Earth Syst. Sci., 22, 2881–2890, https://doi.org/10.5194/hess-22-2881-2018, 2018.
Benettin, P., Nehemy, M. F., Cernusak, L. A., Kahmen, A., and McDonnell, J. J.: On the use of leaf water to determine plant water source: A proof of concept, Hydrol. Process., 35, e14073, https://doi.org/10.1002/hyp.14073, 2021.
Berry, Z. C., Evaristo, J., Moore, G., Poca, M., Steppe, K., Verrot, L., Asbjornsen, H., Borma, L. S., Bretfeld, M., Herve-Fernandez, P., Seyfried, M., Schwendenmann, L., Sinacore, K., Wispelaere, L. D., and McDonnell, J.: The two water worlds hypothesis: addressing multiple working hypotheses and proposing a way forward, Ecohydrology, 11, e1843, https://doi.org/10.1002/eco.1843, 2017.
Bottinga, Y. and Craig., H.: Oxygen isotope fractionation between CO2 and water, and the isotopic composition of marine atmospheric CO2, Earth Planet. Sc. Lett., 5, 285–295, 1969.
Bowen, G. J.: Isoscapes: Spatial pattern in isotopic biogeochemistry, Annu. Rev. Earth Planet. Sci., 38, 161–187, 2010.
Bowen, G. J. and Good, S. P.: Incorporating water isoscapes in hydrological and water resource investigations, Wiley Interdisciplin. Rev.: Water, 2, 107–119, 2015.
Bowen, G. J. and Revenaugh, J.: Interpolating the isotopic composition of modern meteoric precipitation, Water Resour. Res., 39, 1299, https://doi.org/10.1029/2003WR002086, 2003.
Brooks, J. R., Barnard, H. R., Coulombe, R., and McDonnell, J. J.: Ecohydrologic separation of water between trees and streams in a Mediterranean climate, Nat. Geosci., 3, 100–104. 2010.
Cernusak, L. A., Farquhar, G. D., and Pate, J. S.: Environmental and physiological controls over oxygen and carbon isotope composition of Tasmanian blue gum, Eucalyptus globulus, Tree Physiol., 25, 129–146, 2005.
Cernusak, L. A., Barbour, M. M., Arndt, S. K., Cheesman, A. W., English, N. B., field, T. S., Helliker, B. R., Holloway-Phillips, M. M., Holtum, J. A. M., Kahmen, A., McInerney, F. A., Munksgaard, N. C., Simonin, K. A., Song, X., Stuart-Williams, H., West, J. B., and Farquhar, G. D.: Stable isotopes in leaf water of terrestrial plants, Plant Cell Environ., 39, 1087–1102, 2016.
Cernusak, L. A., Barbeta, A., Bush, R., Eichstaedt R., Ferrio, J., Flanagan, L., Gessler, A., Martín-Gómez, P., Hirl, R., Kahmen, A., Keitel., C., Lai, C., Munksgaard, N., Nelson, D., Ogée J., Roden, J., Schnyder, H., Voelker, S., Wang L., Stuart-Williams, H., Wingate, L., Yu, W., Zhao, L., and Cuntz, M.: Do 2H and 18O in leaf water reflect environmental drivers differently?, New Phytol., 235, 41–51, https://doi.org/10.1111/nph.18113, 2022.
Chen, Y., Helliker, B. R., Tang, X., Li, F., Zhou, Y., and Song, X.: Stem water cryogenic extraction biases estimation in deuterium isotope composition of plant source water, P. Natl. Acad. Sci. USA, 117, 33345–33350, 2020.
Chiang, J. C., Fung, I. Y., Wu, C. -H., Cai, Y., Edman, J. P., Liu, Y., Day, J. A., Bhattacharya, T., Mondal, Y., and Labrousse, C. A.: Role of seasonal transitions and westerly jets in East Asian paleoclimate, Quaternary Sci. Rev., 108, 111–129, 2015.
Craig, H. and Gordon, L. I.: Deuterium and oxygen-18 variations in the ocean and the marine atmosphere, in: Proceedings of a conference on stable isotopes in oceanographic studies and paleotemperatures, Pisa, Italy, 9–130, 1965.
Cuntz, M., Ogée, J., Farquhar, G. D., Peylin, P., and Cernusak, L. A.: Modelling advection and diffusion of water isotopologues in leaves, Plant Cell Environ. 30, 892–909, 2007.
Dansgaard, W.: Stable isotopes in precipitation, Tellus, 16, 436–468, 1964.
Dawson, T. E. and Ehleringer, J. R.: Streamside trees that do not use stream water, Nature, 350, 335–337, 1991.
Dongmann. G., Nurnberg, H. E., Forstel, H., and Wagener, K.: On the enrichment of in the leaves of transpiring plants, Radiat. Environ. Biophys., 11, 41–52, 1974.
Draxler, R. R. and Rolph, G. D.: HYSPLIT (Hybrid Single-Particle Lagrangian Integrated Trajectory) Model Access via NOAA ARLREADY.html, NOAA Air Resources Laboratory, http://www.arl.noaa.gov/ready/hysplit4 (last access: 10 April 2021), 2003.
Ehleringer, J. R. and Dawson, T. E.: Water uptake by plants: perspectives from stable isotope composition, Plant Cell Environ., 15, 1073–1082, 1992.
Ellsworth, P. Z. and Williams, D. G.: Hydrogen isotope fractionation during water uptake by woody xerophytes, Plant Soil, 291, 93–107, 2007.
Evaristo, J., Jasechko, S., and McDonnell, J. J.: Global separation of plant transpiration from groundwater and streamflow, Nature, 525, 91–94, 2015.
Farquhar, G. D. and Cernusak, L. A.: On the isotopic composition of leaf water in the non- steady state, Funct. Plant Biol., 32, 293–303, 2005.
Farquhar, G. D. and Gan, K. S.: On the progressive enrichment of the oxygen isotopic composition of water along leaves, Plant Cell Environ., 26, 801–819, 2003.
Farquhar, G. D. and Lloyd, J.: Carbon and oxygen isotope effects in the exchange of carbon dioxide between terrestrial plants and the atmosphere, in: Stable Isotopes and Plant Carbon–Water Relations, edited by: Ehleringer, J. R., Hall, A. E., and Farquhar, G. D., Academic Press, San Diego, 47–70, https://doi.org/10.1016/B978-0-08-091801-3.50011-8, 1993.
Farquhar, G. D., Cernusak, L. A., and Barnes, B.: Heavy water fractionation during transpiration, Plant Physiol., 143, 11–18, 2007.
Gan, K. S., Wong, S. C., Yong, J. W. H., and Farquhar, G. D.: Evaluation of models of leaf water 18O enrichments of spatial patterns of vein xylem, leaf water and dry matter in maize leaves, Plant Cell Environ., 26, 1479–1495, 2003.
Goldsmith, G. R., Munoz-Villers, L. E., Holwerda, F., McDonnell, J. J., Asbjornsen, H., and Dawson, T. E.: Stable isotopes reveal linkages among ecohydrological processes in a seasonally dry tropical montane cloud forest, Ecohydrology, 5, 779–790, 2012.
Helliker, B. R. and Ehleringer, J. R.: Establishing a grassland signature in veins: 18O in the leaf water of C3 and C4 grasses, P. Natl. Acad. Sci. USA, 97, 7894–7898, 2000.
Hepp, J., Schäfer, I. K., Lanny, V., Franke, J., Bliedtner, M., Rozanski, K., Glaser, B., Zech, M., Eglinton, T. I., and Zech, R.: Evaluation of bacterial glycerol dialkyl glycerol tetraether and 2H–18O biomarker proxies along a central European topsoil transect, Biogeosciences, 17, 741–756, https://doi.org/10.5194/bg-17-741-2020, 2020.
Kahmen, A., Sachse, D., Arndt, S. K., Tu, K. P., Farrington, H., Vitousek, P. M., and Dawson, T. E.: Cellulose δ18O is an index of leaf-to-air vapor pressure difference (VPD) in tropical plants, P. Natl. Acad. Sci. USA, 108, 1981–1986, 2011.
Leaney, F., Osmond, C., Allison, G., and Ziegler, H.: Hydrogen-isotope composition of leaf water in C3 and C4 plants: its relationship to the hydrogen-isotope composition of dry matter, Planta, 164, 215–220, 1985.
Lehmann, M. M., Gamarra, B., Kahmen, A., Siegwolf, R. T. W., and Saurer, M.: Oxygen isotope fractionations across individual leaf carbohydrates in grass and tree species, Plant Cell Environ., 40, 1658–1670, 2017.
Li, Z., Feng, Q., Wang, Q., Kong, Y., Cheng, A., Yong, S., Li, Y., Li, J., and Guo, X.: Contributions of local terrestrial evaporation and transpiration to precipitation using δ18O and D-excess as a proxy in Shiyang inland river basin in China, Global Planet. Change, 146, 140–151, 2016.
Li, Z., Li, Z., Yu, H., Song, L., and Ma, J.: Environmental significance and zonal characteristics of stable isotope of atmospheric precipitation in arid Central Asia, Atmos. Res., 227, 24–40, 2019.
Lin, G. H. and Sternberg, L. S. L.: Hydrogen isotopic fractionation by plant roots during water uptake in coastal wetland plants. Stable Isotopic and Plant Carbon/Water Relations, Academic Press, New York, 497–510, https://doi.org/10.1016/B978-0-08-091801-3.50041-6, 1993.
Liu, J.: Seasonality of the altitude effect on leaf wax n-alkane distributions, hydrogen and carbon isotopes along an arid transect in the Qinling Mountains, Sci. Total Environ., 778, 146272, https://doi.org/10.1016/j.scitotenv.2021.146272, 2021.
Liu, J., Liu, W., and An, Z.: Insight into the reasons of leaf wax δDn-alkane values between grasses and woods, Sci. Bull., 60, 549–555, 2015.
Liu, J., Liu, W., An, Z., and Yang, H.: Different hydrogen isotope fractionations during lipid formation in higher plants: Implications for paleohydrology, Sci. Rep., 6, 19711, https://doi.org/10.1038/srep19711, 2016.
Liu, J., Wu, H., Cheng, Y., Jin, Z., and Hu, J.: Stable isotope analysis of soil and plant water in a pair of natural grassland and understory of planted forestland on the Chinese Loess Plateau, Agr. Water Manage., 249, 106800, https://doi.org/10.1016/j.agwat.2021.106800, 2021a.
Liu, J., An, Z., and Lin, G.: Intra-leaf heterogeneities of hydrogen isotope compositions in leaf water and leaf wax of monocots and dicots, Sci. Total Environ., 770, 145258, https://doi.org/10.1016/j.scitotenv.2021.145258, 2021b.
Liu, J., Jiang, C., Guo, L., and Hu, J.: Ecohydrological separation in a pair catchments covered with natural grassland and planted forestland on the Chinese Loess Plateau: Evidence from a one-year stable isotope observation, Hydrol. Process., 36, e14778, https://doi.org/10.1002/hyp.14778, 2022.
Majoube, M.: Fractionnement en oxygen-18 et en deuterium entre l'eau et sa vapeur, Journal de Chimie et Physique, 68, 1423–1436, 1971.
McGuire, K. and McDonnell, J. J.: Stable isotope tracers in watershed hydrology, in: Stable Isotopes in Ecology and Environmental Science, Ecological Methods and Concepts Series, Wiley, 334–374, https://doi.org/10.1002/9780470691854.ch11, 2007.
Munksgaard, N. C., Cheesman, A. W., English, N. B., Zwart, C., Kahmen, A., and Cernusak, L. A.: Identifying drivers of leaf water and cellulose stable isotope enrichment in Eucalyptus in northern Australia, Oecologia, 183, 31–43, 2017.
Ogée, J., Cuntz, M., Peylin, P., and Bariac, T.: Non-steady-state, non-uniform transpiration rate and leaf anatomy effects on the progressive stable isotope enrichment of leaf water along monocot leaves, Plant Cell Environ., 30, 367–387, 2007.
Pagani, M., Pedentchouk, N., Huber, M., Sluijs, A., Schouten, S., Brinkhuis, H., Damsté, J. S. S., and Dichens, G. R.: Arctic hydrology during global warming at the Palaeocene/Eocene thermal maximum, Nature, 442, 671–675, 2006.
Penna, D. and van Meerveld, H. J.: Spatial variability in the isotopic composition of water in small catchments and its effect on hydrograph separation, WIREs Water, 6, e1367, https://doi.org/10.1002/wat2.1367, 2019.
Phillips, S. L. and Ehleringer, J. R.: Limited uptake of summer precipitation by big tooth maple (Acer grandidentatum Nutt) and Gambels oak (Quercus gambelii Nutt), Trees, 9, 214–219, 1995.
Plavcová, L., Hronková, M., Šimková, M., Květoň, J., Vráblová, M., Kubásek, J., Šantrůček, J.: Seasonal variation of δ18O and δ2H in leaf water of Fagus sylvatica L. and related water compartments, J. Plant Physiol., 227, 56–65, 2018.
Poca, M., Coomans, O., Urcelay, C., Zeballos, S. R., Bodé, S., and Boecks, P.: Isotope fractionation during root water uptake by Acacia caven is enhanced by arbuscular mycorrhizas, Plant Soil, 441, 485–497, 2019.
Rienecker, M. M., Suarez, M. J., Gelaro, R., Todling, R., Bacmeister, J., Liu, E., Bosilovich, M. G., Schubert, S. D., Takacs, L., and Kim, G.-K.: MERRA: NASA's modern-era retrospective analysis for research and applications, J. Climate, 24, 3624–3648, 2011.
Romero, I. C. and Feakins, S. I.: Spatial gradients in plant leaf wax D/H across a coastal salt marsh in southern California, Org. Geochem., 42, 618–629, 2011.
Rothfuss, Y. and Javaux, M.: Reviews and syntheses: Isotopic approaches to quantify root water uptake: a review and comparison of methods, Biogeosciences, 14, 2199–2224, https://doi.org/10.5194/bg-14-2199-2017, 2017.
Sachse, D., Billault, I., Bowen, G. J., Chikaraishi, Y., Dawson, T. E., Feakins, S. J., Freeman, K. H., Magill, C. R., McInerney, F. A., van der Meer, M. T. J., Polissar, P. J., Robins, R. J., Sachs, J. P., Schmidt, H. L., Sessions, A. L., White, J. W. C., West, J. B., and Kahmen, A.: Molecular paleoyhydrology: interpreting the hydrogen-isotopic composition of lipid biomarkers from photosynthesizing organisms, Annu. Rev. Earth Planet. Sci., 40, 221–249, 2012.
Šantrůček, J., Kvĕtoň, J., Šetlík, J., and Bulíčková, L.: Spatial variation of deuterium enrichment in bulk water of snowgun leaves, Plant Physiol., 143, 88–97, 2007.
Schefuß, E., Kuhlmann, H., Mollenhauer, G., Prange, M., and Pätzold, J.: Forcing of wet phases in Southeast Africa over the past 17,000 year, Nature, 480, 22–29, 2011.
Song, X., Loucos, K. E., Simonin, K. A., Farquhar, G. D., and Barbour, M. M.: Measurements of transpiration isotopologues and leaf water to assess enrichment models in cotton, New Phytol., 206, 637–646, 2015.
Sprenger, M., Leistert, H., Gimbel, K., and Weiler, M.: hydrological processes at the soil-vegetation-atmosphere interface with water stable isotopes, Rev. Geophys., 54, 674–704, 2016.
Sprenger, M., Tetzlaff, D., and Soulsby, C.: Soil water stable isotopes reveal evaporation dynamics at the soil–plant–atmosphere interface of the critical zone, Hydrol. Earth Syst. Sci., 21, 3839–3858, https://doi.org/10.5194/hess-21-3839-2017, 2017.
Wang, J., Fu, B., Lu, N., and Zhang, L.: Seasonal variation in water uptake patterns of three plant species based on stable isotopes in the semi-arid Loess Plateau, Sci. Total Environ., 609, 27–37, 2017.
Wang, J., Lu, N., and Fu, B.: Inter-comparison of stable isotope mixing models for determining plant water source partitioning, Sci. Total Environ., 666, 685–693, 2019.
Wu, H., Li, J., Li, X., He, B., Liu, J., Jiang, Z., and Zhang, C.: Contrasting response of coexisting plant′s water-use patterns to experimental precipitation manipulation in an alpine grassland community of Qinghai Lake watershed, China, PLoS One, 13, e0194242, https://doi.org/10.1371/journal.pone.0194242, 2018.
Wu, H., Wu, J., Sakiev, K., Liu, J., Li, J., He, B., Liu, Y., and Shen, B.: Spatial and temporal variability of stable isotopes (δ18O and δ2H) in surface waters of arid, mountainous Central Asia, Hydrol. Process., 33, 1658–1669, 2019.
Wu, H., Huang, Q., Fu, C., Song, F., Liu, J., and Li, J.: Stable isotope signatures of river and lake water from Poyang Lake, China: Implications for river-lake interactions, J. Hydrol., 592, 125619, https://doi.org/10.1016/j.jhydrol.2020.125619, 2021.
Zhang, H., Cheng, H., Cai, Y., Spötl, C., Sinha, A., Kathayat, G., and Li, H.: Effect of precipitation seasonality on annual oxygen isotopic composition in the area of spring persistent rain in southeastern China and its paleoclimatic implication, Clim. Past, 16, 211–225, https://doi.org/10.5194/cp-16-211-2020, 2020.
Zhang, H., Zhang, X., Cai, Y., Sinha, A., Spötl, C., Baker, J., Kathayat, G., Liu, Z., Zhao, J., Jia, X., Du, W., Ning, Y., An, Z., Edwards, R. L., and Cheng, H.: A data-model comparison pinpoints Holocene spatiotemporal pattern of East Asian summer monsoon, Quaternary Sci. Rev., 261, 106911, https://doi.org/10.1016/j.quascirev.2021.106911, 2021.
Zhang, P. and Liu, W.: Effect of plant life form on relationship between δD values of leaf wax n-alkanes and altitude along Mount Taibai, China, Org. Geochem., 42, 100–107, 2010.
Zhao, L., Wang, L., Cernusak, L. A., Liu, X., Xiao, H., Zhou, M., and Zhang, S.: Significant difference in hydrogen isotope composition between xylem and tissue water in Populus Euphratica, Plant Cell Environ., 39, 1848–1857, 2016.
Zhao, Y., Wang, Y., He, M., Tong, Y., Zhou, J., Guo, X., Liu, J., and Zhang, X.: Transference of Robinia pseudoacacia water-use patterns from deep to shallow soil layers during the transition period between the dry and rainy seasons in a waterlimited region, Forest Ecol. Manage., 457, 117727, https://doi.org/10.1016/j.foreco.2019.117727, 2020.