the Creative Commons Attribution 4.0 License.
the Creative Commons Attribution 4.0 License.
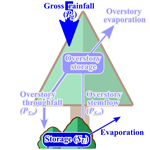
Rainfall interception and redistribution by a common North American understory and pasture forb, Eupatorium capillifolium (Lam. dogfennel)
D. Alex R. Gordon
Miriam Coenders-Gerrits
Brent A. Sellers
S. M. Moein Sadeghi
John T. Van Stan II
In vegetated landscapes, rain must pass through plant canopies and litter to enter soils. As a result, some rainwater is returned to the atmosphere (i.e., interception, I) and the remainder is partitioned into a canopy (and gap) drip flux (i.e., throughfall) or drained down the stem (i.e., stemflow). Current theoretical and numerical modeling frameworks for this process are almost exclusively based on data from woody overstory plants. However, herbaceous plants often populate the understory and are the primary cover for important ecosystems (e.g., grasslands and croplands). This study investigates how overstory throughfall (PT,o) is partitioned into understory I, throughfall (PT) and stemflow (PS) by a dominant forb in disturbed urban forests (as well as grasslands and pasturelands), Eupatorium capillifolium (Lam., dogfennel). Dogfennel density at the site was 56 770 stems ha−1, enabling water storage capacities for leaves and stems of 0.90±0.04 and 0.43±0.02 mm, respectively. As direct measurement of PT,o (using methods such as tipping buckets or bottles) would remove PT,o or disturb the understory partitioning of PT,o, overstory throughfall was modeled () using on-site observations of PT,o from a previous field campaign. Relying on modeled , rather than on observations of PT,o directly above individual plants means that significant uncertainty remains with respect to (i) small-scale relative values of PT and PS and (ii) factors driving PS variability among individual dogfennel plants. Indeed, PS data from individual plants were highly skewed, where the mean per plant was 36.8 %, but the median was 7.6 % (2.8 %–27.2 % interquartile range) and the total over the study period was 7.9 %. PS variability (n=30 plants) was high (CV > 200 %) and may hypothetically be explained by fine-scale spatiotemporal patterns in actual overstory throughfall (as no plant structural factors explained the variability). The total was 71 % (median per gauge was 72 %, with a 59 %–91 % interquartile range). Occult precipitation (mixed dew and light rain events) occurred during the study period, revealing that dogfennel can capture and drain dew to their stem base as PS. Dew-induced PS may help explain dogfennel's improved invasion efficacy during droughts (as it tends to be one of the most problematic weeds in the improved grazing systems in the southeastern US). Overall, dogfennel's precipitation partitioning differed markedly from the site's overstory trees (Pinus palustris), and a discussion of the limited literature suggests that these differences may exist across vegetated ecosystems. Thus, more research on herbaceous plant canopy interactions with precipitation is merited.
- Article
(8198 KB) - Full-text XML
-
Supplement
(1151 KB) - BibTeX
- EndNote
Precipitation (Pg) across most of the global land surface will interact with plant canopies. Precipitation–canopy interactions during storms result in three general hydrologic processes; one which returns water to the atmosphere (interception) and two others that route water to the surface (throughfall and stemflow). Interception is the evaporation of droplets splashing against (Dunkerley, 2009) or stored on canopy surfaces, like leaves (Pereira et al., 2016), bark (Van Stan et al., 2017a) and epiphytes (Porada et al., 2018). Depending on the vegetation and storm conditions, interception can be small per unit area (David et al., 2006) or return half the annual precipitation to the atmosphere (Alavi et al., 2001). In this way, canopy interception can evaporatively cool regions (Davies-Barnard et al., 2014), recycle moisture to generate nearby storms (van der Ent et al., 2014) and reduce stormwater runoff to save millions of dollars (US) in stormwater infrastructure costs (Nowak et al., 2020). Throughfall is the water that drips to the surface through gaps or from canopy surfaces, whereas stemflow is the water that drains down plant stems. The portion of precipitation that drains as throughfall versus stemflow is also highly variable depending on vegetation and storm conditions: ranging annually from 10 % to 90 % for throughfall and from <1 % to 60 % for stemflow (Sadeghi et al., 2020). As throughfall and stemflow reach the surface at different locations, they differentially interact with subsurface hydrological and biogeochemical processes – having been implicated in fine-scale patterns in soil physicochemistry (Gersper and Holowaychuk, 1971), microbial community composition (Rosier et al., 2015, 2016), N-cycling functional genes (Moore et al., 2016) and metazoan community composition (Ptatscheck et al., 2018). Accurate accounting for each of these precipitation partitioning fluxes is, therefore, necessary for the accurate prediction of atmospheric and surface hydro-biogeochemical processes.
Current theoretical and numerical modeling frameworks for canopy precipitation partitioning (see review by Muzylo et al., 2009) are almost exclusively based on observations beneath woody plants, like forests and shrublands (Sadeghi et al., 2020). In forests, the past 150 years of research has primarily targeted dominant overstory trees (Ebermayer, 1873; Van Stan and Gordon, 2018). However, herbaceous plants commonly dominate forest understories and can be abundant beneath shrublands (Jiménez-Rodríguez et al., 2020; Lajtha and Schlesinger, 1986; Specht and Moll, 1983). As a result, our current understanding of “net” precipitation (as measured beneath woody overstory canopies) is not representative of the actual precipitation that reaches the surface (or litter layer; Gerrits and Savenije, 2011) beneath the understory. Herbaceous canopies are relevant to precipitation partitioning in more than the one-third of the global land surface represented by forests; they also cover 27 % and 11 % of the global land surface in grasslands and croplands, respectively (Alexandratos and Bruinsma, 2012; Suttie et al., 2005). It is unlikely that current knowledge on precipitation partitioning based on woody vegetation is applicable to herbaceous vegetation, as they differ in many hydrologically relevant morphological features: smaller height, the lack of bark structure and the presence of other stem features (like trichome hairs or desiccated leaves), among others. This raises unanswered and under-researched, questions that must be addressed to incorporate herbaceous plants in precipitation partitioning theory, including the following:
-
How do these significant morphological differences affect canopy and stem water storage capacities?
-
Do herbaceous plants also favor throughfall generation, like woody plants, or do they more efficiently drain precipitation to their stem bases (and, thereafter, their shallow roots)?
In fact, several long-standing (and hitherto unanswered) calls for greater research on the precipitation partitioning of nonwoody plants (rooted in detailed observations) have been made (Price et al., 1997; Price and Watters, 1989; Verry and Timmons, 1977; Yarie, 1980). These are general questions identified by the community; however, in this study we focus on the following research question: how is overstory throughfall (PT,o: Fig. 1) partitioned into understory interception, throughfall (PT: Fig. 1) and stemflow (PS: Fig. 1) by a dominant forb in disturbed urban forest understories (as well as grasslands and pasturelands), Eupatorium capillifolium (Lam., dogfennel)?
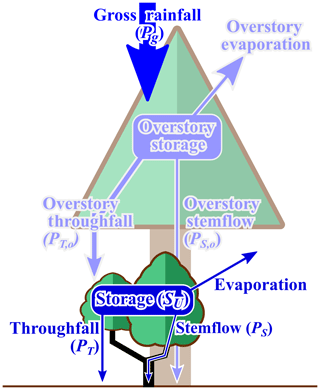
Figure 1Partitioning of gross rainfall by the overstory (light blue) and the understory (dark blue). Overstory throughfall (PT,o), the input to the understory canopy, was estimated from past work at the site. In this study, overstory throughfall was modeled (, see Sect. 2.2.2), and maximum understory water storage capacity (SU), throughfall (PT) and stemflow (PS) were measured.
Very little is known about how understory plants partition PT,o into understory PT and PS (Fig. 1). Overstory stemflow is currently assumed to bypass the understory and litter layers (Carlyle-Moses et al., 2018); however, this assumption, particularly regarding the bypass of litter, has rarely been tested (Friesen, 2020), and overstory stemflow has been observed to runoff for long distances away from the stem (Cattan et al., 2009; Keen et al., 2010). We do not investigate interactions between the understory and overstory stemflow in this study, because stemflow from this study site is negligible (<0.2 %: Yankine et al., 2017). Most observations of precipitation partitioning beneath any plant besides overstory woody plants have been done on maize (Zheng et al., 2019, and references therein) and other cash crops (Drastig et al., 2019, and references therein), which leave plants of forest understories, grasslands or pasturelands relatively unstudied. Even the few studies on forest understory interception, PT, and PS overwhelmingly focus, again, on woody plants (González-Martínez et al., 2017; Price and Watters, 1989), limiting net precipitation observations beneath understory herbaceous plants to ferns (Verry and Timmons, 1977) and nonvascular plants (Price et al., 1997). These scant observations, however, indicate that precipitation partitioning by nonwoody understory plants is hydrologically relevant, as they can store as much water as woody plants (Klamerus-Iwan et al., 2020), evaporate significant portions of PT,o (Coenders-Gerrits et al., 2020) and redistribute 7 %–90 % of event PT,o as PS (Sadeghi et al., 2020). For our study on dogfennel, we hypothesized that, compared with past research on woody plants, dogfennel stems and leaves (i) can store a hydrologically relevant amount of rainwater (i.e., within the range of water storage capacities reported for woody plants; Klamerus-Iwan et al., 2020), (ii) significantly reduce net rainfall flux to the surface (i.e., ) and (iii) redistribute a substantial portion of PT,o to the surface via PS (i.e., PS will often “funnel” more rainwater per storm to the soils surrounding stems than PT, PT,o or Pg over the same area). To test these hypotheses, PT,o was modeled from past on-site observations () as monitoring PT,o, PS and PT simultaneously was not possible without disrupting or removing PT,o. We explicitly acknowledge that the decision to rely on modeled leaves a nontrivial uncertainty regarding the influence of actual overstory throughfall spatiotemporal patterns on small-scale values of PT and individual plants' PS.
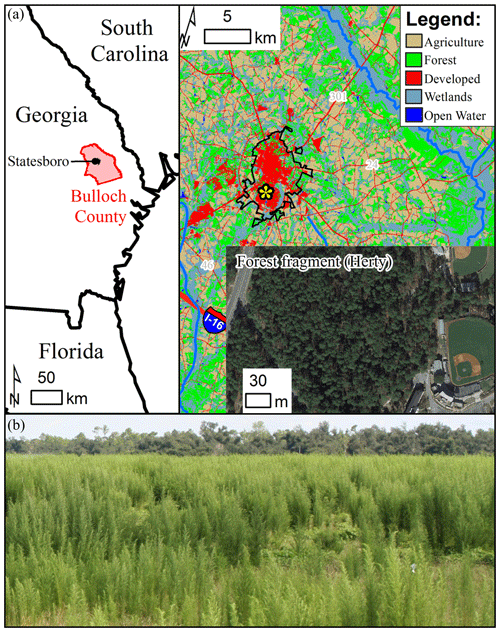
Figure 2(a) Location of the studied Pinus palustris (longleaf pine) forest fragment, Charles H. Herty Pines Nature Preserve, on the Statesboro, Georgia (USA), campus of Georgia Southern University, where Eupatorium capillifolium (dogfennel) is a dominant understory plant. (b) Dogfennel can dominate pastures as well, as shown by the photograph (credit: Brent A. Sellers). The map layers were sourced from state and county boundaries and aerial imagery ©Esri, TomTom North America, Inc. The land use layer was derived from the National Land Cover Database 2011 (full metadata and data access link: https://gdg.sc.egov.usda.gov/Catalog/ProductDescription/NLCD.html, last access: 22 July 2019).
2.1 Study site and study plant description
The study site, the Charles H. Herty Pines Nature Preserve, is a forest fragment in Statesboro, Georgia, USA (Fig. 2a), at Georgia Southern University's main campus (32.430∘ N, 81.784∘ W; 65 m a.s.l.). The climate is subtropical (Köppen Cfa) with mean monthly temperatures (1925–2014) in July that range from 21 to 33 ∘C and generally mild winter months, i.e., the lowest mean January temperature is 3.5 ∘C (University of Georgia, 2019). Mean annual precipitation is 1170 mm yr−1, and precipitation occurs almost exclusively as rain, which is relatively evenly spread over the year. The overstory is dominated by Pinus palustris (longleaf pine), and overstory rainfall partitioning for this site has been reported (Mesta et al., 2017; Van Stan et al., 2018; Yankine et al., 2017). The trunk diameter at breast height (DBH) was relatively consistent across all trees in the study plot: 49.7 cm (mean) with an interquartile range of 36.2–55.7 cm. The mean tree height was 30.4±4.5 m and was derived from terrestrial lidar (terrestrial lidar methods identical to Van Stan et al., 2017a). The stand density was 223 trees ha−1 with 50.4 m2 ha−1 of basal area. Dogfennel, our study plant, was particularly dominant along the forest edge. Dogfennel is a forb of the Asteraceae family that is native to (and widespread across) North America (Van Deelen, 1991; Wunderlin and Hansen, 2003). Although dogfennel behaves as an annual plant throughout much of its North American range, it can behave as a perennial in the southern US by overwintering as a rosette, typically from January to March, before regrowing from a taproot in the spring, typically in April (Macdonald et al., 1992, 1994). Dogfennel can be abundant in disturbed forest understories, particularly pine forests (Brockway et al., 1998) and pastures (Fig. 2b). In the study pine forest, the dogfennel stem density was 56 770 stems ha−1 along the stand edge. In pasturelands, dogfennel can reach this stem density within a single season and, if left unmanaged, dogfennel densities have been measured as high as 74 stems m−2, or ∼740 000 stems ha−1 (Dias et al., 2018). The growth habit of dogfennel results in “clumps” of stems. The dogfennel density was estimated in ten 10 m × 10 m plots by counting the stems per clump for three randomly selected clumps in each plot. For each plot, the mean stems per clump were multiplied by the number of clumps per plot. Finally, all stems per plot were summed and scaled to 1 ha. Three dogfennel clumps were randomly selected for throughfall and stemflow monitoring. Within these three clumps, 30 individual dogfennel stems were randomly selected for stemflow monitoring. Individual plant attributes – canopy radius (cm), stem radius (cm), leaf angle at the stem (degrees from vertical) at various canopy heights (1.00, 1.25, 1.50, 1.75, 2.00 m), and the relative location within the clump, interior (I), middle (M) or exterior (E) – were measured for each stemflow-instrumented plant (Table 1). Canopy and stem radii were determined manually with a tape measure, where canopy radii were the mean of measurements from eight directions (N, NE, E, SE, S, SW, W and NW) and stem radius was determined by a single manual measurement at the stem base. The leaf angle at the stem was determined for two leaves at each height using the Protractor™ app for iPhone (2013, Phoenix Solutions) which logs an angle after the leveling of the iPhone camera (see Fig. S1 in the Supplement for an example).
2.2 Hydrometeorological monitoring
2.2.1 Rainfall measurements
Rainfall amount, duration and intensity for discrete rain events were automatically logged every 5 min by a weather station installed above the canopy (on the rooftop of nearby Brannen Hall at a height of ∼40 m), which is located 100 m from Charles H. Herty Pines Nature Preserve. Rainfall observations were recorded by three tipping bucket gauges (TE525MM, Texas Electronics, Dallas, TX, USA) interfaced with a CR1000 datalogger (Campbell Scientific, Logan, Utah, USA). This weather station logged a suite of other meteorological variables; however, as these data do not represent the meteorological conditions experienced by the understory, they are not reported or examined here. A discrete event was defined as any atmospheric moisture (rainfall or dew) that resulted in a measurable quantity of throughfall and stemflow (more than a few milliliters) that occurred after a minimum inter-storm dry period of 8 h. Few events consisted of early morning dew contributions (visually observed during sampling and verified by air temperatures equalling dew point temperatures), and these occurred after low-magnitude nighttime rainfall. When dew was present in the understory, there was no response from above-canopy rain gauges; thus, a post hoc estimate of occult dew contribution to PT,o was made by assuming the dew contribution was equal to the understory canopy water storage capacity (1.33 mm – methods described later). An important limitation to this dew estimate is that it represents the maximum possible dew contribution. Rain events without dewfall required at least ∼4 mm of rainfall for generation of PT or PS from the monitored dogfennel canopies.
2.2.2 Overstory throughfall estimation
As observing PT,o directly would prevent direct observation of PT and PS beneath dogfennel plants, PT,o was estimated from previous field measurements at the site (Fig. 3). Automated PT,o monitoring was performed from September 2016 to September 2017 using ten 3.048 m long and 10.16 cm diameter PVC troughs oriented at a moderate slope, with a 5.08 cm slot cut lengthwise for collection and drainage of PT,o to a Texas Electronics (Dallas, Texas, USA) TR-525I tipping bucket gauge, resulting in a 1.65 m2 collection area. Tipping bucket gauges and their associated troughs were randomly placed within a 0.25 ha plot and recorded every 5 min by a CR1000 datalogger. All trough angles were measured with a digital clinometer to correct computations of the trough area receiving PT,o. Trough and tipping bucket assemblies were field tested to ensure accuracy (±5 %) under storm conditions typical for the region (Van Stan et al., 2016). These PT,o data were reported by Mesta et al. (2017). To estimate overstory throughfall, , a regression model was generated from the association between PT,o (% of rainfall) measured on site and storm size, and R (millimeters per storm) using the “Aston” curve (Aston, 1979):
where a and b are regression coefficients. This model and its fit statistics are provided in Fig. 3. We assume that the past observed rainfall relationship with PT,o at the site was similar during our study period. Although we are unable to assess if and the degree to which there is a difference between these observation periods, the canopy is mature and there has been no known or noticeable disturbance or change in canopy structure since the previous observation period.
2.2.3 Understory throughfall and stemflow measurements
Throughfall gauges consisted of nine randomly placed funnels (506.7 cm2 collection area each), three per dogfennel clump (1520.1 cm2 total collection area per clump), connected to high-density polyethylene (HDPE) bottles that were manually measured with graduated cylinders immediately after a storm ended (within 4 h). The total canopy area of dogfennel plants at this site rarely exceed 2000 cm2; thus, the total throughfall gauge area per clump generally represented >75 % of canopy area, which is a comparatively much larger gauge-to-canopy area than most past throughfall studies on forest canopies (Van Stan et al., 2020).
Standard stemflow measurement methods developed for woody plants (use of flexible tubing wrapped around a woody stem; Sadeghi et al., 2020) are not suitable for dogfennel; moreover, no standard stemflow collection devices exist for herbaceous plants. Thus, stemflow collars were constructed from aluminum foil, 15 mm inner-diameter flexible polyethylene tubing, electrical tape and silicon (see Fig. S2). The aluminum foil was folded over itself several times to strengthen the collar (typically a ∼160 mm length of foil was folded to ∼40 mm) and connected to plastic tubing with stainless steel staples. The aluminum collar was then folded around the lower stem of the dog fennel and secured with electrical tape. To seal the aluminum foil, staple connections, and the interstices between the foil, tubing and stem, silicon was thinned with hydro-treated light (95 %–100 %) naphtha (VM&P Naphtha, Klean-Strip, Memphis, TN, USA), allowing for it to completely fill the aluminum cone up to the tube opening and make a watertight seal. While naphtha-thinned silicon was poured into collars, the tube opening was covered. An additional benefit of naphtha-thinned silicon was that, due to the evaporation of naphtha, the silicon shrinks, pulling the collar taut and stiffening and strengthening the stemflow collection device and extending the lifespan of the collar. Stemflow was measured with a graduated pipette (with 1 mL graduations) from 500 mL plastic bottles connected to the tubing base.
2.3 Water storage capacity estimation
Maximum water storage capacity, Su (mm), was estimated for the dogfennel canopy and stem, both as volume (L) per unit surface area (m2). All field leaf and stem samples were collected during an inter-storm dry period (>24 h after any rainfall). For the canopy, 50 leaves representing the median size of the site dogfennel plants were sampled (broken off at the base of the leaf), taken back to the lab, their “field-dry” mass (g) was determined on a bench scale and then the broken ends of their leaf-stems were sealed with silicon to prevent water exchange from an area that was not previously exposed in its natural state. Sampling for the stems was similar; however, as dogfennel heights reach (and can exceed) 2 m, the stems were cut into 5 cm sections. Just as with the leaves, 50 representative samples of these stem sections were weighed in the lab and then sealed with silicon on both ends. Next, all leaf samples and stem sections were submerged in water for 3 d until maximum saturation was achieved (per Van Stan et al., 2015), whereupon the maximum saturation mass (g) was recorded. For comparison with the field-dry mass, all samples were oven-dried until their mass no longer changed (mass recorded every 3 h), whereupon the oven-dried mass (g) was recorded. No leaf or stem samples were oven dried longer than 15 h. The gravity convection oven (Isotemp, Fisher Scientific) was set to 40 ∘C (confirmed with a standard thermometer). The maximum volume of all samples' water storage capacity is the difference between the saturation and oven-dried masses. The oven-dried leaves and stems did not visually appear to be damaged (aside from the sampling cuts, obviously), and care was taken to ensure the plant samples were not damaged. It is likely that internal (not externally intercepted) water was exchanged during this process; however, this is not entirely problematic as plant surfaces are known to permit interaction between externally intercepted water and internal water (Berry et al., 2019). Moreover, we explicitly acknowledge that although these submersion methods are commonly used, they produce the “maximum” possible water storage capacity (hence, our objective to estimate maximum water storage capacity), as multiple intrinsic and extrinsic factors of plant surfaces could reduce the available water storage capacity in situ (Klamerus-Iwan et al., 2020).
Specific water storage capacity, SL (mL cm−2), for the leaves and stems was determined by dividing the lab-derived maximum volume (mL) by the samples' surface area (cm2). For leaves, after sampling, leveled photos of each sample were taken on a grid system (every block representing 2.5 cm × 2.5 cm for scale), and the leaf images were then vectorized and processed for 2-D projected surface area using the “Measure Path” extension in Inkscape (v. 0.92, Inkscape.org). An example vectorized image of leaf area is provided in the Supplement (Fig. S3). Error in this vector-based leaf surface area estimate was estimated by repeating the process five times for each leaf. Stem surface area for all samples was estimated from their radii and height. SL estimates for the stem (0.436 mL cm−2) and leaves (0.195 mL cm−2) were then scaled to SU (mm as L m−2) using stem and leaf surface area estimates per plant (A=171.9 and 807.5 cm2 per plant, respectively), and multiplied by the site plant density (D=5.68 plants m−2) before being divided by 1000:
Plant stem and leaf surface area estimates were determined from five representative plants that were cut from the site and separated into leaves and stems, and the sums of the leaf and stem areas (determined as mentioned earlier in the paragraph) were then divided by 5. Total leaf surface area compares well to values reported from ∼1 m tall dogfennel plants (212 cm2 per plant; Carlisle et al., 1980) considering that our plants were much taller (∼2 m).
2.4 Data analysis
Descriptive statistics were compiled for all variables presented and regression analyses were performed to relate plant canopy and hydrologic variables. All statistical analyses were done using Statistica 12 (StatSoft, Tulsa, OK, USA). Throughfall volumes (L) from all gauges were summed and converted to yields (mm) by dividing by the total gauge area (m2). Stemflow yield (mm) for an individual plant was determined by dividing its volume (L) by the projected canopy area (m2). To compare stemflow production across plants, two metrics were computed per plant for each storm: normalized stemflow, (–), and the funneling ratio, F (–). was computed per Keim et al. (2005):
where PS,i is stemflow volume (mL) from each individual plant in a single storm, is the mean stemflow for all plants in a single storm and sS is the standard deviation of stemflow for all plants in a single storm. F values for individual plants in each storm were computed per (Herwitz, 1986):
where Bi is the basal area (cm2) at the base of an individual plant, and P will be either Pg or (this will be explicitly indicated in the results). There are an increasing number of F metrics (Carlyle-Moses et al., 2018; Levia and Germer, 2015); however, the selected method is the most common F metric applied to stemflow data to date. Moreover, in situ observations of non-collared dogfennel plants during rainfall confirmed that dogfennel PS rates did not produce visible runoff areas.
3.1 Storm and plant structural conditions
Discrete rain events, as measured above the forest canopy, ranged in magnitude from 0.1 mm (during dewfall) to 101.3 mm (Table 1). The distribution of storm magnitudes was skewed, such that the mean, 16.5 mm, was many times greater than the median, 6.6 mm (Table 1). Estimated overstory throughfall (), as shown in Fig. 3, ranged from 0 (again, during dewfall) to 72.2 mm, with a median of 3.5 mm (Table 1). Thirty of the plants in the selected dogfennel clusters – those being monitored for stemflow – had an average canopy radius of 18.3 cm (±4.5 cm standard deviation), which was nearly identical to the median canopy radius (Table 1). The stem radii of all measured dogfennel plants ranged from 0.1 to 0.7 cm, with a mean radius of 0.6 cm (Table 1). The resulting ratio of canopy : stem radii was also normally distributed, with a mean and median of ∼36 (dimensionless), but ranged from 24 to 50 (Table 1). For all plants, the mean leaf angle decreased from 54 to 32∘ from vertical with increasing canopy height, i.e., the higher in the dogfennel canopy, the closer the leaf angle was to vertical (Table 1). This trend appears consistent across each individual study plant regardless of which clump the plants' were located in, as the standard deviation across all elevations are low, 1.8–3.1∘ from vertical, and do not overlap (Table 1).
Table 2Descriptive statistics of relative throughfall (PT) and stemflow (PS) yield from dogfennel plants expressed as a proportion of gross rainfall (Pg) and modeled overstory throughfall (). Coefficients of variation (CV) and quartile variation (CQV) are also provided. For storms where dew occurred in the understory, dew was not measured by above-canopy Pg gauges but was included in the estimate by assuming that dew represented at least an additional 1.33 mm (i.e., Su).
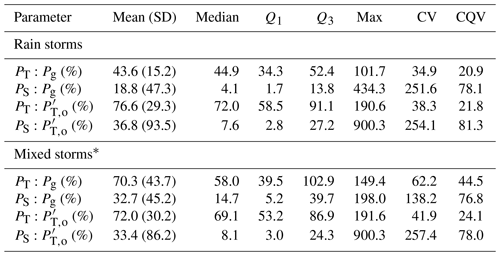
* Storms with occult precipitation.
3.2 Partitioning into water storage, throughfall and stemflow
Note that is an event-scale estimate derived from past observations, limiting its utility in examining fine-scale PT and individual-plant scale PS. The sum of data from all storms throughout the study period resulted in PT, PS and I of 71 %, 8 % and 21 % as a portion of , respectively, beneath dogfennel plants at our site. Water storage capacity achieved by dogfennel leaves in the lab was 0.90±0.04 mm, whereas dogfennel stems stored a capacity of 0.43±0.02 mm (Fig. 4). This resulted in the total SU of dogfennel plants in the understory of this study site being approximately 1.3 mm. This SU estimate agrees with the reductions of below dogfennel plants; for example, mean was 76.6 % for rain-only storms (Table 2), or a mean yield of PT=12.9 mm which exceeds a 1.3 mm reduction (due to SU and evaporation) in the estimated mean yield, 16.5 mm (from Table 1). A large portion of the rainwater captured on dogfennel canopies was able to overcome the stem water storage capacity and generate PS. Dogfennel PS data were highly skewed, producing a mean relative PS () of 36.8 % but a median of 7.6 % within a narrow interquartile range, 2.8 %–27.2 % (Table 2). For events including occult precipitation, both maximum and exceeded 100 %: during mixed storms reached a maximum at 192 %, whereas the maximum for was just over 900 % (Table 2). Note that dew in the understory was not measured by the above-canopy rainfall gauges, and was only increased by an assumed maximum dew contribution equal to SU (1.33 mm); thus, dew accumulation allows PT and PS to exceed 100 % of Pg and (Table 2). When compared to rainfall above the overstory (Pg), the medians are much smaller: PT:Pg values are 45 % and 58 % for rain-only storms and mixed storms, respectively, and PS:Pg values are 4.1 % and 14.7 %, respectively (Table 2).
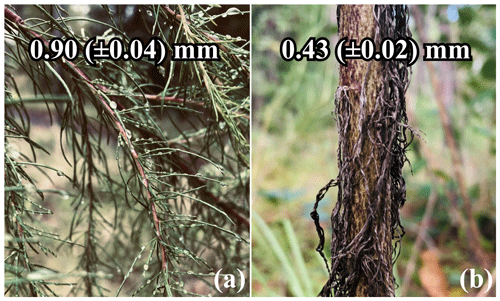
Figure 4Water storage capacity (standard error) for the (a) canopy and (b) stem of Eupatorium capillifolium (dogfennel) per lab-based submersion tests on samples collected from the Charles H. Herty Pines Nature Preserve understory.
Yield values (mm) were estimated for dogfennel PT and PS across storms, and both event-level PT and PS yields linearly correlated with estimated event-level (Fig. 5a, b). Regarding PT, as the catchment area (canopy area above the gauge) is equal to the input area (soil area below the gauge), the PT yield from the canopy and the PT supply to the surface are equal; therefore, the term “yield” will be applied for both. Median PT yield beneath dogfennel for the measured storms was 4.4 mm with an interquartile range of 1.1 to 11.3 mm (Fig. 5c). The maximum PT yield approached 50 mm during a large-magnitude rain storm (where Pg=101.3 mm). As the canopy area that generates stemflow is many times greater than the surface area around plant stems that receive stemflow (see Table 1), PS yield and F will differ. F values are typically used to represent PS supply to soils. Yields of PS from dogfennel were as high as 24 mm, but the median was 0.4 mm and the interquartile range was narrow, 0.1–1.3 mm (Fig. 5c).
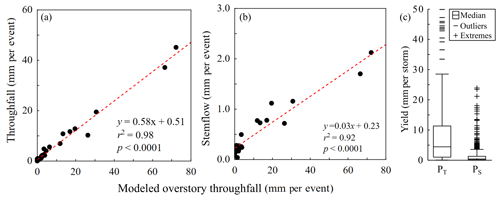
Figure 5Scatter plots showing the response of Eupatorium capillifolium (dogfennel) (a) throughfall (PT) and (b) stemflow (PS) yields across all rainfall events (without occult precipitation). (c) Boxplot showing yields from individual PT gauges and plants' PS. The line and box represent the median and interquartile range, respectively, and the whiskers represent the non-outlier range; other symbols represent outliers and extreme values.
3.3 Stemflow and throughfall variability
Coefficients of variability (CV) and quartile variability (CQV) were computed for both PS and PT, relative to Pg and (Table 2), and storm-normalized temporal stability plots were generated for PS yield only (Fig. 6). Storm-normalized temporal stability plots were not generated for PT yields because the experimental design accounts for the spatial variability of PT via the deployment of large gauge areas (compared with the dogfennel canopy area); this permits estimates of variability across a few large-area gauges (Table 2), but it limits the observable variability. CV and CQV for relative PT ranged from 22 % to 90 % and were generally lower for rain-only storms, <40 %, than for mixed storms, >60 % (Table 2). Variability in relative PS across study plants, ranging from 77 % to 257 %, was always greater than observed for relative PT for the monitored storms (Table 2). Due to the greater skew in the relative PS data compared with relative PT, the CV was many times greater than CQV for relative PS (Table 2). CV and CQV for was similar for rain and the mixed storms; however, the CV for PS:Pg was greater for rain-only storms compared with mixed storms.
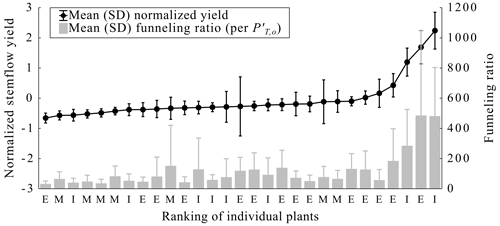
Figure 6Mean and standard deviation (SD) of normalized stemflow yield per plant and the associated funneling ratio per Herwitz (1986) and using modeled overstory throughfall () in order of rank per mean normalized stemflow yield. Plant locations within clusters are indicated as follows: E denotes external; M denotes middle, between the interior and exterior; and I denotes interior.
Temporal stability of normalized stemflow, (Fig. 6) indicates that there were only a few plants that captured most of the PT,o drained as stemflow (three plants' mean ). Thus, most of the studied dogfennel plants captured similar amounts of as stemflow – having between −1 and 1 (y=0 represents the central tendency of data). Funneling ratios (F based on ) show that all plants concentrated PS yields to the surface around their stem bases (Fig. 6). Mean F across all plants was 87, and for the 27 plants whose mean fell between −1 and 1, median F ranged from 18 to 200 (Fig. 6). However, for the three plants with the highest , their mean F values were 287, 476 and 484 (Fig. 6). These voluminous stemflow-generating plants single-handedly account for one-third of total PS volume (8734 from 27 870 mL). To evaluate possible canopy structural influences on PS variability, various directly measured structural metrics were compared: radii of canopies and stems and the vertical variability in leaf angle (see Fig. S4). No clear visible or statistical correlations or correspondences were found between these structural variables and across plants (Fig. S4). In fact, variability in the measured canopy structural variables was low (Table 1) compared with the variability observed for dogfennel PS and (Fig. 6).
4.1 Overstory throughfall partitioning by dogfennel
Partitioning of overstory throughfall by this example dominant understory and pasture forb resulted in hydrologically relevant losses of rainwater to the surface at our site (Table 2). As the maximum water storage capacity is a major driver of rainfall interception (Klaassen et al., 1998), the magnitude of dogfennel's overstory throughfall interception may be attributed to its canopy being able to store a sizable magnitude of rainwater per unit area, 1.33 mm (Fig. 4). Although mass changes of dried and submerged vegetation samples are discrepant from the processes and temporal scales of natural rainfall interception, it is a common method with well-known and long-discussed limitations that was selected to estimate water storage capacity as more direct water storage capacity estimation methods are still currently under development – see discussions in reviews by Friesen et al. (2015) and Klamerus-Iwan et al. (2020). Methodological limitations withstanding, the SU estimates in this study fit within the range of water storage capacities of other herbaceous plants synthesized by Breuer et al. (2003). This synthesis is focused on the leaves of herbaceous plants (alongside other plant types) (Breuer et al., 2003), but less research has estimated the stem component (or reported a total including the stem component) of the water storage capacity for short vegetation (Bradley et al., 2003; Wang et al., 2016; Wohlfahrt et al., 2006; Yu et al., 2012). The stems of herbaceous plants, even thick smooth stems (>1 cm in diameter) can store nearly 0.5 mm, e.g., Taraxacum officinale (dandelion) (Wohlfahrt et al., 2006). Even thin (<1 cm radius) herbaceous stems with epidermal outgrowths, like hairs, can store large amounts of rainwater, e.g., 0.25 mm for Achillea millefolium (yarrow) and 0.20 mm for Trifolium pretense (red clover) (Wohlfahrt et al., 2006). In the case of dogfennel stem water storage capacity at our site, the 0.43 mm estimate is within this range, and its magnitude is likely a result of two principal factors: (1) dense stem coverage by desiccated leaves (photo in Fig. 4) and (2) the fact that this species can achieve large densities, up to 700 000 stems ha−1 (Dias et al., 2018) – 56 770 stems ha−1 at our study site. We note that, to our knowledge, stem water storage capacities for herbaceous plants with spines, thorns and other such physical structures have not been evaluated.
Overstory throughfall was also redistributed into a highly spatially variable (Table 2) but temporally persistent pattern beneath dogfennel canopies (where CV or CQV was approximately 20 %–40 % for PT and 80 %–250 % for PS; Table 2), despite all measured canopy structures – such as branch angle, stem size and canopy size – being similar (Table 1). As our sampling plan measured PT over a large area of the dogfennel canopy (rather than at numerous localized points), this discussion point will focus on the intraspecific PS observations. The high spatial variability and temporal persistence of PS across plants despite canopy structural similarity raises the following question: what caused the intraspecific PS patterns observed in this study? A likely explanation may be that, in this case, access to precipitation for stemflow production is related to overstory throughfall patterns (which, we reiterate, were not able to be measured without removing or disrupting PT and PS). Overstory throughfall patterns are known to be spatially variable but temporally persistent across forest types (Van Stan et al., 2020). Specifically, individual dogfennel plants that persistently generated greater PS than other plants may have just received greater overstory throughfall from persistent overstory drip points. If the overstory throughfall pattern is a major driver of intraspecific variability in PS in this study, then the funneling ratios computed from mean overstory throughfall (per Fig. 3) would be incorrect (in Fig. 6). In this case, funneling ratios (computed from the localized overstory throughfall above each plant) could be similar across the monitored dogfennel plants. Testing this hypothesized relationship between dogfennel PS patterns and overstory throughfall patterns was not possible in the field, as sampling overstory throughfall would prevent PS from being generated by the plant. Future work to test this hypothesis could, however, make use of rainfall simulators.
The large diversion of rainwater and dew to their stem base may be partially responsible for dogfennel survival during extended periods of drought (or improved invasion efficacy during droughts; Loveless, 1959; Forthman, 1973), and may also explain why this species tends to be one of the most problematic in improved grazing systems located in Florida (Sellers et al., 2009). Rainfall patterns in central and south Florida may also intersect with dogfennel's canopy water balance to “tip the scales” in its favor. Specifically, rainfall in our study region is often limited from January through May, with the bulk of the rainfall occurring from June through October, and the water storage capacity of burgeoning dogfennel plants during early spring may enhance the chances of individual plant survival (resulting in large infestations as referenced previously).
4.2 Overstory (woody) and understory (herbaceous) canopies may partition rainfall differently
The dominant understory plant at our study site, dogfennel, intercepted similar amounts of modeled overstory throughfall, with an interquartile range of 11 %–59 % per storm (Table 2), compared to the gross rainfall interception by their overstory pine canopy, which had an interquartile range of 19 %–60 % per storm (Van Stan et al., 2017b). Similar rainwater interception between dogfennel and the pine overstory may be due to dogfennel's maximum water storage capacity comparing favorably to that of overstory tree species, 0.07–4.30 mm (Klamerus-Iwan et al., 2020). Even the maximum stem water storage capacity is of a similar magnitude to values reported by past work on woody plants, 0.2–5.9 mm (Klamerus-Iwan et al., 2020), albeit at the lower end of the range. Most current research on stem water storage has focused on intrinsic factors of woody plant stems, like bark thickness, porosity, microrelief or roughness (Ilek et al., 2017; Levia and Herwitz, 2005; Levia and Wubbena, 2006; Sioma et al., 2018; Van Stan et al., 2016; Van Stan and Levia, 2010); however, other stem structures besides bark may be capable of storing substantial water, e.g., the desiccated leaves of our study plant.
There were differences in how gross rainfall was redistributed by the overstory canopy compared with how modeled overstory throughfall was redistributed by the dogfennel understory. Stemflow from the overstory, P. palustris was negligible at this site, 0.2 % of gross rainfall (Yankine et al., 2017), but median dogfennel PS was 7.6 % of modeled overstory throughfall (with an interquartile range of 2.8 %–27.2 %) (Table 2). Annual relative PS (and PT) estimates from trees and herbaceous plants reported by previous work indicates that herbaceous plants are generally greater stemflow producers than woody plants (Sadeghi et al., 2020). Although relative PT beneath dogfennel was similar to observations of relative overstory throughfall beneath P. palustris at this site (Mesta et al., 2017), throughfall has been found to be generally lower beneath herbaceous plant canopies than for woody plant canopies (Sadeghi et al., 2020). This seems reasonable because if interception is similar between herbaceous plants and woody plants, an increase in relative stemflow would necessitate a decrease in relative throughfall. The results of this study support statements by several past studies suggesting that plants in the understory and overstory interact differently with rainfall. Thus, we repeat the long-standing calls for increased research on understory precipitation partitioning, particularly stemflow (Price et al., 1997; Price and Watters, 1989; Verry and Timmons, 1977; Yarie, 1980).
4.3 A brief discussion on dew-generated throughfall and stemflow
For a few storms (n=5), dew contributed significantly to PT and PS by the studied dogfennel plants. The median PT generated from dew beneath dogfennel plants at our site was 0.74 mm per plant with an interquartile range of 0.47–0.99 mm per plant, resulting in a total dew-related contribution to PT of 17.1 mm over the study period. Volumes of stemflow under dewfall totaled 558 mL for all study plants, with individuals supplementing the dew-related PT with up to 61 mL per plant (yielding an additional ∼0.6 mm). Dew contributions to net precipitation below plant canopies have rarely been studied. The earliest quantity for dew drainage was 0.08 mm from a single event on a single tree in Johanniskreuz, Germany (Ney, 1893). Since then, to our knowledge, only one other study has examined dew-related drainage from plants, focusing on stemflow from the herbaceous Ambrosia artemisiifolia, or common ragweed (Shure and Lewis, 1973). They estimated that the drainage of dew via PS resulted in an additional input of 1.1 L per month during the growing season and hypothesized that this process may “play a vital role in governing the density, diversity, and distribution of plant species within field ecosystems” (Shure and Lewis, 1973). Dew drainage from plant canopies and down stems may, in addition to being a valuable water source, influence plant–soil interactions by transporting leached or dry-deposited materials to the soils – something also discussed by Shure and Lewis (1973). Globally, dew contributes a small percentage to the annual precipitation (Baier, 1966); however, in semiarid and arid (Baier, 1966; Hao et al., 2012), as well as summer-dry climates (Tuller and Chilton, 1973), dew can form a significant water input. In such ecologic settings as these, it is, therefore, reasonable to suppose that any factor that doubles the frequency of plant-moisture availability, even though the amounts be small, must materially affect the plant growing condition. Thus, further research is needed to assess dew (and mixed storm) drainage in arid and semiarid climates, with days on which dew occurs being ≥70 % yr−1 (Hao et al., 2012). The global importance of occult precipitation and resulting wet canopy conditions has recently been reviewed and described as a critical future research direction for plant sciences (Dawson and Goldsmith, 2018). Given these scant but ecologically relevant findings, further research on the influence of condensation events on plant–soil interactions via throughfall and stemflow may be merited.
Eupatorium capillifolium (Lam., dogfennel) in the understory of an urban forest fragment intercepted 21 % of modeled overstory throughfall from Pinus palustris (Mill.). The remaining 71 % and 8 % of modeled overstory throughfall reached the surface beneath dogfennel plants as understory throughfall and stemflow, respectively. At the stand scale, the partitioning of modeled overstory throughfall by this understory forb differs considerably from the rainfall partitioning of the woody overstory, especially regarding stemflow (7.9 % versus <0.2 %). During a few storms that occurred in tandem with dewfall, dogfennel plants were able to augment stemflow (and throughfall) production by capturing dew. These processes may help explain how dogfennel survives extended droughts and even shows improved invasion efficacy during droughts, making it one of the most problematic weeds in southeastern US grazing systems. Stemflow variability among individual plants was very high (CV ∼ 250 %), but no dogfennel canopy structures measured in this study provided statistically significant insights into this stemflow variability. Future work will assess the extent to which actual overstory throughfall variability drives understory stemflow variability for plants, like dogfennel, of similar intraspecific canopy structure. The inability to measure fine-scale overstory throughfall patterns without disturbing understory rainfall partitioning in the field is a nontrivial limitation of this study – a limitation that future work may overcome with rainfall simulations. Still, in forests, overstory throughfall is not the final frontier for determining net rainfall, and investigations on how it is intercepted and redistributed by herbaceous plants is needed to improve our understanding of exactly how much (and in what pattern) rainfall reaches the surface. For other vegetated ecosystems where herbaceous plants are the overstory (grasslands and croplands), precipitation partitioning research is also needed.
Data are permanently archived at https://digitalcommons.georgiasouthern.edu/ (last access: September 2020) (Georgia Southern University, 2020) and freely available.
The supplement related to this article is available online at: https://doi.org/10.5194/hess-24-4587-2020-supplement.
DARG conceived and designed the study in consultation with JTVS and AMJCG. DARG designed field collection devices in consultation with JTVS and AMJCG, deployed the devices, collected data, performed the data analysis, and drafted the initial article with input from all authors. BAS contributed expertise regarding relevant rangeland and pastureland topics and assisted with data analysis and interpretation. SMMS performed a literature synthesis for discussions comparing herbaceous and woody plants' rainfall partitioning and used this synthesis to assist in paper writing. JTVS was the principal undergraduate research supervisor for DARG. All authors contributed to revisions of the paper.
The authors declare that they have no conflict of interest.
This article is part of the special issue “Water, isotope and solute fluxes in the soil–plant–atmosphere interface: investigations from the canopy to the root zone”. It is not associated with a conference.
The authors thank Georgia Southern University's Division of Facilities Services for study site access, maintenance and security. We also gratefully acknowledge the rigorous, thoughtful and helpful comments of the reviewers.
This research has been supported by the U.S. Department of Education (Ronald E. McNair Postbaccalaureate Achievement Program) and the Nederlandse Organisatie voor Wetenschappelijk Onderzoek (grant no. 863.12.022).
This paper was edited by Natalie Orlowski and reviewed by two anonymous referees.
Alavi, G., Jansson, P.-E., Hällgren, J.-E., and Bergholm, J.: Interception of a dense spruce forest, performance of a simplified canopy water balance model, Hydrol. Res., 32, 265–284, 2001.
Alexandratos, N. and Bruinsma, J.: World agriculture towards 2030/2050: the 2012 revision, ESA Working paper, FAO, Rome, 2012.
Aston, A. R.: Rainfall interception by eight small trees, J. Hydrol., 42, 383–396, 1979.
Baier, W.: Studies on dew formation under semi-arid conditions, Agricult. Meteorol., 3, 103–112, 1966.
Berry, Z. C., Emery, N. C., Gotsch, S. G., and Goldsmith, G. R.: Foliar water uptake: processes, pathways, and integration into plant water budgets, Plant Cell Environ., 42, 410–423, 2019.
Bradley, D. J., Gilbert, G. S., and Parker, I. M.: Susceptibility of clover species to fungal infection: the interaction of leaf surface traits and environment, Am. J. Bot., 90, 857–864, 2003.
Breuer, L., Eckhardt, K., and Frede, H.-G.: Plant parameter values for models in temperate climates, Ecol. Model., 169, 237–293, 2003.
Brockway, D. G., Wolters, G. L., Pearson, H. A., Thill, R. E., Baldwin, V. C., and Martin, A.: Understory plant response to site preparation and fertilization of loblolly and shortleaf pine forests, J. Range Manage., 51, 47–54, 1998.
Carlisle, R. J., Watson, V. H., and Cole, A. W.: Canopy and chemistry of pasture weeds, Weed Sci., 28, 139–141, 1980.
Carlyle-Moses, D. E., Iida, S. I., Germer, S., Llorens, P., Michalzik, B., Nanko, K., Tischer, A., and Levia, D. F.: Expressing stemflow commensurate with its ecohydrological importance, Adv. Water Resour., 121, 472–479, 2018.
Cattan, P., Ruy, S. M., Cabidoche, Y. M., Findeling, A., Desbois, P., and Charlier, J. B.: Effect on runoff of rainfall redistribution by the impluvium-shaped canopy of banana cultivated on an Andosol with a high infiltration rate, J. Hydrol., 368, 251–261, 2009.
Coenders-Gerrits, A., Schilperoort, B., and Jiménez-Rodríguez, C.: Evaporative Processes on Vegetation: An Inside Look, in: Precipitation Partitioning by Vegetation: A Global Synthesis, chap. 3, edited by: Van Stan, J. T., Gutmann, E., and Friesen, J., Springer Nature, 35–48, https://doi.org/10.1007/978-3-030-29702-2_3, 2020.
David, T. S., Gash, J. H. C., Valente, F., Pereira, J. S., Ferreira, M. I., and David, J. S.: Rainfall interception by an isolated evergreen oak tree in a Mediterranean savannah, Hydrol. Process., 20, 2713–2726, https://doi.org/10.1002/hyp.6062, 2006.
Davies-Barnard, T., Valdes, P., Jones, C., and Singarayer, J.: Sensitivity of a coupled climate model to canopy interception capacity, Clim. Dynam., 42, 1715–1732, 2014.
Dawson, T. E. and Goldsmith, G. R.: The value of wet leaves, New Phytol., 219, 1156–1169, 2018.
Dias, J. L., Sellers, B. A., Ferrell, J. A., Silveira, M. L., and Vendramini, J.: Herbage Responses to Dogfennel Cover and Limited Nitrogen Fertilization in Bahiagrass Pastures, Agron. J., 110, 2507–2512, https://doi.org/10.2134/agronj2018.02.0084, 2018.
Drastig, K., Quiñones, T. S., Zare, M., Dammer, K.-H., and Prochnow, A.: Rainfall interception by winter rapeseed in Brandenburg (Germany) under various nitrogen fertilization treatments, Agr. Forest Meteorol., 268, 308–317, 2019.
Dunkerley, D. L.: Evaporation of impact water droplets in interception processes: Historical precedence of the hypothesis and a brief literature overview, J. Hydrol., 376, 599–604, https://doi.org/10.1016/j.jhydrol.2009.08.004, 2009.
Ebermayer, E.: Physical Effects of Forests on Air and Soil and their Climatological and Hygienic Importance, Krebs, Aschaffenburg, Germany, 1873.
Forthman, C. A.: The effects of prescribed burning on sawgrass, Cladium jamaicense Crantz, South Florida, MS thesis, University of Miami, Coral Gables, FL, USA, 1973.
Friesen, J.: Flow Pathways of Throughfall and Stemflow through the Subsurface, in: Precipitation Partitioning by Vegetation: A Global Synthesis, chap. 13, edited by: Van Stan, J. T., Gutmann, E., and Friesen, J., Springer Nature, Cham, 215–228, https://doi.org/10.1007/978-3-030-29702-2_13, 2020.
Friesen, J., Lundquist, J., and Van Stan, J. T.: Evolution of forest precipitation water storage measurement methods, Hydrol. Process., 29, 2504–2520, https://doi.org/10.1002/hyp.10376, 2015.
Georgia Southern University: Digital Commons @ Georgia Southern, available at: https://digitalcommons.georgiasouthern.edu/, last access: September 2020.
Gerrits, A. and Savenije, H.: Forest floor interception, in: Forest Hydrology and Biogeochemistry, chap. 22, Springer, Dordrecht, 445–454, https://doi.org/10.1007/978-94-007-1363-5_22, 2011.
Gersper, P. L. and Holowaychuk, N.: Some effects of stem flow from forest canopy trees on chemical properties of soils, Ecology, 52, 691–702, 1971.
González-Martínez, T. M., Williams-Linera, G., and Holwerda, F.: Understory and small trees contribute importantly to stemflow of a lower montane cloud forest, Hydrol. Process., 31, 1174–1183, https://doi.org/10.1002/hyp.11114, 2017.
Hao, X. M., Li, C., Guo, B., Ma, J. X., Ayupa, M., and Chen, Z. S.: Dew formation and its long-term trend in a desert riparian forest ecosystem on the eastern edge of the Taklimakan Desert in China, J. Hydrol., 472–473, 90–98, 2012.
Herwitz, S. R.: Infiltration-excess caused by stemflow in a cyclone-prone tropical rainforest, Earth Surf. Proc. Land., 11, 401–412, 1986.
Ilek, A., Kucza, J., and Morkisz, K.: Hygroscopicity of the bark of selected forest tree species, iForest – Biogeosci. Forest., 10, 220–226, https://doi.org/10.3832/ifor1979-009, 2017.
Jiménez-Rodríguez, C. D., Coenders-Gerrits, M., Wenninger, J., Gonzalez-Angarita, A., and Savenije, H.: Contribution of understory evaporation in a tropical wet forest during the dry season, Hydrol. Earth Syst. Sci., 24, 2179–2206, https://doi.org/10.5194/hess-24-2179-2020, 2020.
Keen, B., Cox, J., Morris, S., and Dalby, T.: Stemflow runoff contributes to soil erosion at the base of macadamia trees, in: 19th World Congress of Soil Science, Soil Solutions for a Changing World, 1–6 August 2010, Brisbane, Australia, 240–243, 2010.
Keim, R. F., Skaugset, A. E., and Weiler, M.: Temporal persistence of spatial patterns in throughfall, J. Hydrol., 314, 263–274, https://doi.org/10.1016/j.jhydrol.2005.03.021, 2005.
Klaassen, W., Bosveld, F., and De Water, E.: Water storage and evaporation as constituents of rainfall interception, J. Hydrol., 212, 36–50, 1998.
Klamerus-Iwan, A., Link, T., Keim, R., and Van Stan, J.: Storage and routing of precipitation through canopies, in: Precipitation Partitioning by Vegetation: A Global Synthesis, chap. 2, edited by: Van Stan, J. T., Gutmann, E., and Friesen, J., Springer Nature, Cham, 17–34, https://doi.org/10.1007/978-3-030-29702-2_2, 2020.
Lajtha, K. and Schlesinger, W. H.: Plant response to variations in nitrogen availability in a desert shrubland community, Biogeochemistry, 2, 29–37, 1986.
Levia, D. F. and Germer, S.: A review of stemflow generation dynamics and stemflow-environment interactions in forests and shrublands, Rev. Geophys., 53, 673–714, 2015.
Levia, D. F. and Herwitz, S. R.: Interspecific variation of bark water storage capacity of three deciduous tree species in relation to stemflow yield and solute flux to forest soils, Catena, 64, 117–137, https://doi.org/10.1016/j.catena.2005.08.001, 2005.
Levia Jr., D. F., and Wubbena, N. P.: Vertical variation of bark water storage capacity of Pinus strobus L. (Eastern white pine) in southern Illinois, Northeast. Nat., 13, 131–137, 2006.
Loveless, C. M.: A study of the vegetation in the Florida Everglades, Ecology, 40, 1–9, 1959.
Macdonald, G. E., Brecke, B. J., and Shilling, D. G.: Factors affecting germination of dogfennel (Eupatorium capillifolium) and yankeeweed (Eupatorium compositifolium), Weed Sci., 40, 424–428, 1992.
Macdonald, G. E., Brecke, B. J., Colvin, D. L., and Shilling, D. G.: Chemical and mechanical control of dogfennel (Eupatorium capillifolium), Weed Technol., 8, 483–487, 1994.
Mesta, D., Van Stan, J. T., Yankine, S., Cote, J., Jarvis, M., Hildebrandt, A., Friesen, J., and Maldonado, G.: Canopy rainfall partitioning across an urbanization gradient in forest structure as characterized by terrestrial LiDAR, in: AGU Fall Meeting, December 2017, New Orleans, LA, USA, 2017.
Moore, L. D., Van Stan, J. T., Gay, T. E., Rosier, C., and Wu, T.: Alteration of soil chitinolytic bacterial and ammonia oxidizing archaeal community diversity by rainwater redistribution in an epiphyte-laden Quercus virginiana canopy, Soil Biol. Biochem., 100, 33–41, https://doi.org/10.1016/j.soilbio.2016.05.016, 2016.
Muzylo, A., Llorens, P., Valente, F., Keizer, J. J., Domingo, F., and Gash, J. H. C.: A review of rainfall interception modelling, J. Hydrol., 370, 191–206, https://doi.org/10.1016/j.jhydrol.2009.02.058, 2009.
Ney, C. E.: Der Wald und die Quellen, Tubingen, Verlag von Franz Bießder, Tübingen, p. 101, 1893.
Nowak, D., Coville, R., Endreny, T., Abdi, R., and Van Stan, J. T.: Valuing Urban Tree Impacts on Precipitation Partitioning, in: Precipitation Partitioning by Vegetation: A Global Synthesis, edited by: Van Stan, J. T., Gutmann, E., and Friesen, J., Springer Nature, Cham, Switzerland, 2020.
Pereira, F. L., Valente, F., David, J. S., Jackson, N., Minunno, F., and Gash, J. H.: Rainfall interception modelling: Is the wet bulb approach adequate to estimate mean evaporation rate from wet/saturated canopies in all forest types?, J. Hydrol., 534, 606–615, https://doi.org/10.1016/j.jhydrol.2016.01.035, 2016.
Porada, P., Van Stan, J. T., and Kleidon, A.: Significant contribution of non-vascular vegetation to global rainfall interception, Nat. Geosci., 11, 563–567, 2018.
Price, A., Dunham, K., Carleton, T., and Band, L.: Variability of water fluxes through the black spruce (Picea mariana) canopy and feather moss (Pleurozium schreberi) carpet in the boreal forest of Northern Manitoba, J. Hydrol., 196, 310–323, 1997.
Price, A. G. and Watters, R. J.: The influence of the overstory, understory and upper soil horizons on the fluxes of some ions in a mixed deciduous forest, J. Hydrol., 109, 185–197, 1989.
Ptatscheck, C., Milne, P. C., and Traunspurger, W.: Is stemflow a vector for the transport of small metazoans from tree surfaces down to soil?, BMC Ecol., 18, 43, 2018.
Rosier, C. L., Van Stan, J. T., Moore, L. D., Schrom, J. O. S., Wu, T., Reichard, J. S., and Kan, J.: Forest canopy structural controls over throughfall affect soil microbial community structure in an epiphyte-laden maritime oak stand, Ecohydrology, 8, 1459–1470, https://doi.org/10.1002/eco.1595, 2015.
Rosier, C. L., Levia, D. F., Van Stan, J. T., Aufdenkampe, A., and Kan, J.: Seasonal dynamics of the soil microbial community structure within the proximal area of tree boles: Possible influence of stemflow, Eur. J. Soil Biol., 73, 108–118, https://doi.org/10.1016/j.ejsobi.2016.02.003, 2016.
Sadeghi, S., Gordon, A., and Van Stan, J. T.: A Global Synthesis of Throughfall and Stemflow Hydrometeorology, in: Precipitation Partitioning by Vegetation: A Global Synthesis, chap. 4, edited by: Van Stan, J. T., Gutmann, E., and Friesen, J., Springer Nature, Cham, 49–70, https://doi.org/10.1007/978-3-030-29702-2_4, 2020.
Sellers, B. A., Ferrell, J. A., MacDonald, G. E., and Kline, W. N.: Dogfennel (Eupatorium capillifolium) size at application affects herbicide efficacy, Weed Technol., 23, 247–250, 2009.
Shure, D. J. and Lewis, A. J.: Dew formation and stem flow on common ragweed (Ambrosia artemisiifolia), Ecology, 54, 1152–1155, 1973.
Sioma, A., Socha, J., and Klamerus-Iwan, A.: A New Method for Characterizing Bark Microrelief Using 3D Vision Systems, Forests, 9, 30, 2018.
Specht, R. and Moll, E.: Mediterranean-type heathlands and sclerophyllous shrublands of the world: an overview, in: Mediterranean-type Ecosystems, Springer, Heidelberg, 41–65, https://doi.org/10.1007/978-3-642-68935-2_2, 1983.
Suttie, J. M., Reynolds, S. G., and Batello, C.: Grasslands of the World, Food & Agriculture Org., Rome, 514 pp., 2005.
Tuller, S. E. and Chilton, R.: The role of dew in the seasonal moisture balance of a summer-dry climate, Agricult. Meteorol., 11, 135–142, 1973.
University of Georgia Weather Network: Historical Data, Statesboro, Bulloch County, Georgia, 2019.
Van Deelen, T.: Eupatorium capillifolium, US Department of Agriculture, Forest Service, Rocky Mountain Research Station, Fire Sciences Laboratory, Missoula, MT, USA, 1991.
van der Ent, R. J., Wang-Erlandsson, L., Keys, P. W., and Savenije, H. H. G.: Contrasting roles of interception and transpiration in the hydrological cycle –Part 2: Moisture recycling, Earth Syst. Dynam., 5, 471–489, https://doi.org/10.5194/esd-5-471-2014, 2014.
Van Stan, J. T. and Gordon, D. A.: Mini-Review: Stemflow as a Resource Limitation to Near-Stem Soils, Front. Plant Sci., 9, 248, https://doi.org/10.3389/fpls.2018.00248, 2018.
Van Stan, J. T. and Levia, D. F.: Inter- and intraspecific variation of stemflow production from Fagus grandifolia Ehrh. (American beech) and Liriodendron tulipifera L. (yellow poplar) in relation to bark microrelief in the eastern United States, Ecohydrology, 3, 11–19, https://doi.org/10.1002/eco.83, 2010.
Van Stan, J. T., Stubbins, A., Bittar, T., Reichard, J. S., Wright, K. A., and Jenkins, R. B.: Tillandsia usneoides (L.) L. (Spanish moss) water storage and leachate characteristics from two maritime oak forest settings, Ecohydrology, 8, 988–1004, https://doi.org/10.1002/eco.1549, 2015.
Van Stan, J. T., Gay, T. E., and Lewis, E. S.: Use of multiple correspondence analysis (MCA) to identify interactive meteorological conditions affecting relative throughfall, J. Hydrol., 533, 452–460, https://doi.org/10.1016/j.jhydrol.2015.12.039, 2016.
Van Stan, J. T., Norman, Z., Meghoo, A., Friesen, J., Hildebrandt, A., Côté, J.-F., Underwood, S. J., and Maldonado, G.: Edge-to-Stem Variability in Wet-Canopy Evaporation From an Urban Tree Row, Bound.-Lay. Meteorol., 165, 295–310, 2017a.
Van Stan, J. T., Coenders-Gerrits, M., Dibble, M., Bogeholz, P., and Norman, Z.: Effects of phenology and meteorological disturbance on litter rainfall interception for a Pinus elliottii stand in the Southeastern United States, Hydrol. Process., 31, 3719–3728, https://doi.org/10.1002/hyp.11292, 2017b.
Van Stan, J. T., Underwood, S. J., and Friesen, J.: Urban Forestry: An underutilized tool in water management, in: Advanced Tools for Integrated Water Resources Management, Advances in Chemical Pollution, Environmental Management and Protection, edited by: Friesen, J. and Rodriguez-Sinobas, L., Elsevier, London, UK, 35–62, 2018.
Van Stan, J. T., Hildebrandt, A., Friesen, J., Metzger, J. C., and Yankine, S. A.: Spatial variablity and temporal stability of local net precipitation patterns, in: Precipitation Partitioning by Vegetation: A Global Synthesis, chap. 6, edited by: Van Stan, J. T., Gutmann, E., and Friesen, J., Springer Nature, Cham, 89–104, https://doi.org/10.1007/978-3-030-29702-2_6, 2020.
Verry, E. S. and Timmons, D.: Precipitation nutrients in the open and under two forests in Minnesota, Can. J. Forest Res., 7, 112–119, 1977.
Wang, B., Wu, F., Xiao, S., Yang, W., Justine, M. F., He, J., and Tan, B.: Effect of succession gaps on the understory water-holding capacity in an over-mature alpine forest at the upper reaches of the Yangtze River, Hydrol. Process., 30, 692–703, 2016.
Wohlfahrt, G., Bianchi, K., and Cernusca, A.: Leaf and stem maximum water storage capacity of herbaceous plants in a mountain meadow, J. Hydrol., 319, 383–390, 2006.
Wunderlin, R. P. and Hansen, B. F.: Guide to the vascular plants of Florida, University Press of Florida, Gainesville, FL, USA, 2003.
Yankine, S. A., Van Stan, J. T., Mesta, D. C., Côté, J.-F., Hildebrandt, A., Friesen, J., and Maldonado, G.: What controls stemflow? A LiDAR-based investigation of individual tree canopy structure, neighborhood conditions, and meteorological factors, AGU Fall Meeting, December 2017, New Orleans, LA, USA, 2017.
Yarie, J.: The role of understory vegetation in the nutrient cycle of forested ecosystems in the mountain hemlock biogeoclimatic zone, Ecology, 61, 1498–1514, 1980.
Yu, K., Pypker, T. G., Keim, R. F., Chen, N., Yang, Y., Guo, S., Li, W., and Wang, G.: Canopy rainfall storage capacity as affected by sub-alpine grassland degradation in the Qinghai–Tibetan Plateau, China, Hydrol. Process., 26, 3114–3123, 2012.
Zheng, J., Fan, J., Zhang, F., Yan, S., Wu, Y., Lu, J., Guo, J., Cheng, M., and Pei, Y.: Throughfall and stemflow heterogeneity under the maize canopy and its effect on soil water distribution at the row scale, Sci. Total Environ., 660, 1367–1382, 2019.