the Creative Commons Attribution 4.0 License.
the Creative Commons Attribution 4.0 License.
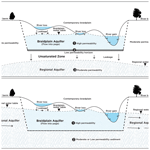
Conceptualising surface water–groundwater exchange in braided river systems
Scott R. Wilson
Jo Hoyle
Richard Measures
Antoine Di Ciacca
Leanne K. Morgan
Eddie W. Banks
Linda Robb
Thomas Wöhling
Braided rivers can provide substantial recharge to regional aquifers, with flow exchange between surface water and groundwater occurring at a range of spatial and temporal scales. However, the difficulty in measuring and modelling these complex and dynamic river systems has hampered process understanding and the upscaling necessary to quantify these fluxes. This is due to an incomplete understanding of the hydrogeological structures that control river–groundwater exchange. In this paper, we present a new conceptualisation of subsurface processes in braided rivers based on observations of the main losing reaches of three braided rivers in Aotearoa / New Zealand.
The conceptual model is based on a range of data, including lidar, bathymetry, coring, particle size distribution, groundwater level and temperature monitoring, radon-222, electrical-resistivity tomography and fibre-optic cables. The combined results indicate that sediments within the recently active river braidplain are distinctive, with sediments that are poorly consolidated and better sorted compared with adjacent deposits from the historical braidplain that become successively consolidated and intermixed with flood silt deposits due to overbank flow.
A distinct sedimentary unconformity, combined with the presence of geomorphologically distinct lateral boundaries, suggests that a “braidplain aquifer” forms within the active river braidplain through the process of sediment mobilisation during flood events.
This braidplain aquifer concept introduces a shallow storage reservoir to the river system, which is distinct from the regional aquifer system, and mediates the exchange of flow between individual river channels and the regional aquifer. The implication of the new concept is that surface water–groundwater exchange occurs at two spatial scales: the first is hyporheic and parafluvial exchange between the river and braidplain aquifer; the second is exchange between the braidplain aquifer and regional aquifer system. Exchange at both scales is influenced by the state of hydraulic connection between the respective water bodies. This conceptualisation acknowledges braided rivers as whole “river systems”, consisting of channels and a gravel aquifer reservoir.
This work has important implications for understanding how changes in river management (e.g. surface water extraction, bank training and gravel extraction) and morphology may impact groundwater recharge (and potentially flow, temperature attenuation and ecological resilience) under dry conditions.
- Article
(12973 KB) - Full-text XML
- BibTeX
- EndNote
This study is motivated by the need to understand processes and quantify losses from braided river systems to alluvial aquifers. In Aotearoa / New Zealand, more than 150 river systems are thought to have braided reaches (Brower et al., 2024) that provide a substantial component of recharge to alluvial aquifers. For example, in the case of the Wairau Aquifer, it has been estimated that recharge is sourced almost exclusively from the Wairau River (Wöhling et al., 2018).
Braided rivers are spatially complex, dynamic hydrologic environments that are not easily measured using field techniques or represented by numerical models. The determination of flow exchanges between the river and groundwater in such a hydrologically complex and morphologically dynamic environment is difficult because of their spatial and temporal flow complexity, numerous and changing number of channels, dynamic bathymetry, coarse substrate, and tendency for loss of field installations during high-flow events. The complexity of this challenge demands a collaborative effort that leverages the strengths of a diverse range of disciplines to develop a comprehensive and holistic understanding of the problem.
Many authors have studied surface–aquifer exchanges, and several reviews have been conducted on available techniques for measuring exchanges for alluvial rivers in general (Kalbus et al., 2006; González-Pinzón et al., 2015; Brunner et al., 2017), ephemeral rivers at different spatial scales (Banks et al., 2011; Shanafield and Cook, 2014), and braided rivers (Coluccio and Morgan, 2019). Based on these reviews, there is a tendency for previous studies to focus on quantifying exchange fluxes prior to understanding the hydrological processes that influence the exchange. Ward and Packman (2019) suggest that, despite the large amount of research on river–groundwater exchanges, an accurate predictive, transferable understanding of the river corridor is lacking. Indeed, a conceptualisation of how braided rivers relate to their underlying groundwater systems is lacking (Coluccio and Morgan, 2019). This has led to ambiguity in what measured exchanges represent as well as difficulty in trying to represent braided rivers within aquifer-scale models.
The aim of this paper is to formulate a conceptualisation of braided river exchange processes at both the local (reach) and sub-catchment (aquifer) scale. This conceptualisation provides a framework that helps provide (1) context for field measurements of flow exchange to be interpreted and (2) potential representation of local-scale processes in sub-catchment models. Incorporating these local-scale processes is vital to predict how changes in the river system can impact groundwater recharge.
The conceptualisation presented here was developed based on field observations; however, for clarity of explanation, we introduce the conceptual framework first and then present the supporting evidence. Our working definition for hyporheic exchange is local bed-scale interaction that occurs within a single channel (e.g. a single riffle), whereas parafluvial exchange occurs between individual channels at larger scales (across a bar or further). We also distinguish between a river, which consists of a series of wetted channels, and a river system, which consists of wetted channels plus subsurface flow through the associated braidplain gravels. “Braidplain” refers to the lateral extent occupied by the river braids, old bar surfaces and abandoned channels (Warburton, 1996; Gray et al., 2016; Brower et al., 2024). The extent of wetted channels and recently reworked bed material (bare gravel) at a given point in time defines the “active braidplain”, which also has potential to shift laterally. Lateral adjustment of the active braidplain may be limited by hillslope margins; terraces; or, in managed rivers, by rock revetments or artificial stop banks (levees), which are typically protected with vegetative buffers. We refer to the extent within which our study rivers can currently adjust as the “contemporary braidplain”, acknowledging that the contemporary braidplain margins in two of our study rivers (Wairau and Ngaruroro) are controlled by engineered flood defences that have narrowed the natural braidplain such that almost the entire contemporary braidplain is active.
The prevailing conceptualisation for gravel-bed rivers generally consists of a surface channel with an associated bed with some hydraulic resistance (Schälchli, 1992; Wu and Huang, 2000) that exchanges water with an associated fluvial or alluvial aquifer (Stanford and Ward, 1993; Poole and Berman, 2001). Within the alluvial aquifer lies a hyporheic zone that functions as an interface between groundwater and surface waters (Stanford and Ward, 1993; Poole and Berman, 2001; Boano et al., 2014). The extent of the hyporheic zone is defined by its function or process of interest, which can be physical, chemical, biological or a combination of functions (Ward, 2015). Accordingly, the vertical or lateral boundaries of the hyporheic zone are transient, flexible and not easily defined spatially (White, 1993; Boulton et al., 1998; Ward, 2015). From a hydrological perspective, the hyporheic zone has been defined as the extent to which surface water enters the high-porosity subsurface beneath and lateral to a stream and returns to the stream surface farther downstream (Harvey and Wagner, 2000). Authors have suggested that the hyporheic zone extends from the upper few centimetres of sediment (Boulton et al., 1998; Sophocleous, 2002) to larger scales (km3), constituting a hyporheic corridor (Stanford and Ward, 1993). Valett et al. (1996) predict the extent of the hyporheic zone to be related to catchment lithology, with interaction being more extensive at sites with higher alluvial hydraulic conductivity, whereas Boano et al. (2008) predict the infiltration depth to be related to bedform for any given hydraulic conductivity.
An additional, ecological concept is that of a riparian zone (Steiger et al., 2005). This extends river margins beyond the active channel to include the biosphere supported by and including recent fluvial landforms and inundated or saturated by bank discharge (Hupp and Osterkamp, 1996). Other authors have considered the presence of a parafluvial zone, situated between the hyporheic zone (beneath the river channel) and riparian zone (Holmes et al., 1994), that accommodates longer flow paths within the alluvium adjacent to the stream (Bourke et al., 2014; Cartwright and Hoffmann, 2016). Our interpretation of the parafluvial zone is that it constitutes exchange flow within the alluvial aquifer at spatial and temporal scales beyond what is considered hyporheic.
From a hydrological perspective of braided rivers, the framework that emerges from the prevailing concepts is one in which river–groundwater exchange occurs within an alluvial aquifer that conveys both hyporheic and parafluvial flow paths. The proportion of these flow path components theoretically depends on the degree to which there is a net loss or gain in river channel flow. However, the interpretation of river–groundwater exchanges becomes challenging in a braided river system comprising multiple channels within an alluvial aquifer. Harvey and Gooseff (2015), Barthel and Banzhaf (2016), and Ward and Packman (2019) propose that exchange fluxes be considered at different spatial scales: point, local, sub-catchment and regional. Following this approach, exchange within individual river braids or channels can be considered to occur at the point scale, whereas the sum of all braids within a river reach can be considered to be a local-scale interaction. While process understanding can be observed and fluxes quantified at the point and local scales, it is imperative to enable an upscaling of observed processes so that fluxes can be estimated for at least the sub-catchment (aquifer) scale.
Previous work on subsurface structure in braided rivers has tended to focus on the role of bed material heterogeneity. A significant body of literature exists to describe braided river deposits via morphology (Huber and Huggenberger, 2016), sedimentology (Huggenberger and Regli, 2006; Theel et al., 2020), geophysics (Pirot et al., 2019) and modelling approaches (Pirot et al., 2014, 2015; Brunner et al., 2017; Schilling et al., 2022). To date, no conceptual model has been posed for how a braided river and its associated braidplain gravels (alluvial aquifer) relate to those of the underlying regional aquifer. While the structural components of river–groundwater interaction have been identified by previous authors (e.g. Poole and Berman, 2001; Steiger et al., 2005), the identification of clear spatial boundaries between structural elements has been missing. From a hydrological perspective of understanding surface water–groundwater interaction, this creates a problem with respect to where the river system ends and where the regional groundwater system begins. The uncertainty related to this lack of spatial definition transfers to the interpretation of field data regarding whether a sample is representative of river channel flow, alluvial aquifer (hyporheic or parafluvial zones) or regional groundwater.
Consequently, representation of braided rivers in numerical models is problematic, as their complexity is not readily captured by a simple conceptualisation. Water exchanges can potentially be simulated realistically using a fully coupled hydrological model such as HydroGeoSphere (Therrien et al., 2010; Brunner and Simmons, 2012). However, the data required to parameterise such a model as well as the computational demands of the detailed mesh required to simulate exchanges in braided rivers make this approach only suitable for point- and local-scale studies. Furthermore, braided river morphology is so dynamic that a new bed morphology would be required following each significant flood event. In recent years, two approaches to simulate the transitions of dynamic bed morphology and sediments in river-groundwater exchanges have been tested. The first approach applied the ensemble Kalman filter and areal imagery to assimilate riverbed topography and to update aquifer hydraulic conductivities in a HydroGeoSphere model for a 2 km section of the Emme River in Switzerland (Tang et al., 2018). The data assimilation scheme strongly improved predictions of post-flood hydraulic states of the system. The second approach proposed a pilot point parameterisation scheme in which both the aquifer properties (hydraulic conductivity) and the location of the pilot points are inferred, e.g. from riverbed training images (Khambhammettu et al., 2020). The corresponding travelling pilot points (TRIPS) scheme could potentially be used to describe the transition between discrete states of river morphology. To some extent, these approaches enable the application of fully integrated 3D models in dynamic river environments of appropriate scale, although their application in a larger river system or at a larger scale is untested.
The simple river structure offered by the Streamflow-Routing (SFR; Niswonger and Prudic, 2005) and River (Harbaugh, 2005) packages in MODFLOW represent the river as a flux boundary condition with vertical flow impedance in the bed expressed by a lumped parameter termed “streambed conductance”. Previous authors have shown that the concept of a streambed resistance concentrating all pressure losses, as implemented in MODFLOW, is questionable in many cases (Anderson, 2005; Rushton, 2007; Morel-Seytoux et al., 2018; Di Ciacca et al., 2019). Moreover, even if such a streambed exists, a major physical issue with a lumped parameter approach is that streambed conductance values in the field are not homogenous but, rather, vary spatially (Cardenas and Zlotnik, 2003; Zhou et al., 2014; Pryshlak et al., 2015; Laube et al., 2018) and temporally (Levy et al., 2011; Wu et al., 2015). In a braided river, bed material typically consists of a heterogeneous mixture of cobbles, gravels and sands, which can have similar characteristics to alluvial sediments located several metres beneath the bed. Therefore, the streambed conductance concept seems inappropriate to represent surface water–groundwater exchange in braided river systems.
Different modelling approaches have been trialled to represent braided rivers in Aotearoa / New Zealand. White et al. (2012) conducted a steady-state water balance approach to determine flow losses for a reach of the Waimakariri River. Exchanges between individual channels and the adjacent alluvial aquifer were determined via mass balance, although the subsurface components of the exchange were not explicitly described. An alternative approach by Wöhling et al. (2018, 2020) simulated dynamic Wairau River exchanges at the sub-catchment scale using MODFLOW. In this case, the braided nature of the river was not considered, and the river was represented by the SFR package using a stage–width–flow relationship derived from a representative channel morphology. While this model fitted river flux and groundwater level data well, the approach employed a streambed conductance model, which is difficult to reconcile with the river morphology and bed sediment seen in the field. A particular drawback of the SFR package is its inability to represent the hyporheic or parafluvial exchange fluxes observed at the point or local scale.
While the Waimakariri and Wairau modelling studies are relatively comprehensive, an understanding of subsurface structure is missing from the river representation in both cases. This lack of knowledge about the structural controls on subsurface flow in the braided river environment needs clarification to understand what measured and modelled river–groundwater exchanges represent. In doing this, a more physically realistic method for representing braided rivers in numerical surface water–groundwater flow models may be achieved.
A conceptual framework is proposed that captures the key elements of water exchanges in a braided river system. This conceptualisation builds on the previous work of Fox and Durnford (2003) and Brunner et al. (2009a, b) and recognises that the hydrological controls on river–groundwater exchange occur at two distinct interfaces within a braidplain system. Specifically, these two exchange processes can be summarised as follows:
-
river channel ↔ braidplain aquifer (hyporheic and/or parafluvial exchange);
-
braidplain aquifer ↔ regional aquifer (river system–groundwater exchange).
The first exchange interface is within the active braidplain, and it occurs between individual river channels (braids) and the local shallow water table in the river bed sediments at the point or local scale. We term the water stored within these river bed sediments the “braidplain aquifer” (BPA), which facilitates hyporheic and parafluvial flow. For a perennially flowing river, the BPA will retain some degree of saturation throughout the year, although unsaturated conditions may occur in the case of intermittent or ephemeral rivers if there are prolonged periods with no river flow. Individual river braids can be in hydraulic connection (gaining or losing), disconnection or a transitional state relative to the BPA. At the active braidplain interface, all possible river exchange processes can occur, regardless of the hydraulic relationship between the BPA and surrounding regional water table. The second exchange interface occurs between the BPA and regional water table at both the local (reach) and sub-catchment (aquifer) scale.
Central to this conceptualisation is the presence of a distinct BPA immediately beneath the river surface that facilitates exchange at these two interfaces. A similar term “braided-river aquifer” has been used by previous authors (Pirot et al., 2015), although we have not found an associated definition. The BPA functions as a storage medium to exchange water between the river and regional aquifer in braided river systems. Direct exchange of water between the river and regional aquifer can only occur if the river channel is in direct contact with the regional aquifer (i.e. where the surface water extends to the boundary of the BPA, for example, where a channel follows the lateral margin of the BPA, or there is a connection at the base of a deep scour pool).
A key feature of the BPA is its extremely high transmissivity, which is a product of the highly dynamic nature of braided rivers. Bedload transport during flood events causes braids to form, migrate and be abandoned; processes that rework the river bed sediments (Bristow and Best, 1993; Reinfelds and Nanson, 1993). This reworking process strips most fine sediment (silts and clays) from the bed of the river, as the shear stresses during floods are too high for these size fractions to be deposited except in vegetated areas and backwaters. The result is a braidplain deposit of high-transmissivity lag gravels. High transmissivity combined with the relatively large bed slope of braided rivers and the presence of multiple braids for water exchange produces a groundwater flow path that is sub-parallel to the contemporary braidplain, regardless of the regional groundwater flow direction.
Conceptual diagrams have been drawn for situations in which the BPA is hydraulically disconnected from (Fig. 1a) and connected to (Fig. 1b) the regional aquifer. The first case (Fig. 1a) consists of three functional lithological layers representing a BPA that is hydraulically disconnected from an underlying regional aquifer by an unsaturated zone. In this case, water moves vertically from the BPA to the regional aquifer under a unit hydraulic gradient. A minimum of three layers is required for an unsaturated zone to develop (Fox and Durnford, 2003), and there must be a sufficient transmissivity contrast between the impeding horizon and underlying sediments (Brunner et al., 2009a, b). It should be noted that braided river deposits are lithologically variable and complex, and only two functional layers are required in most cases due to stratification in the underlying sediments.
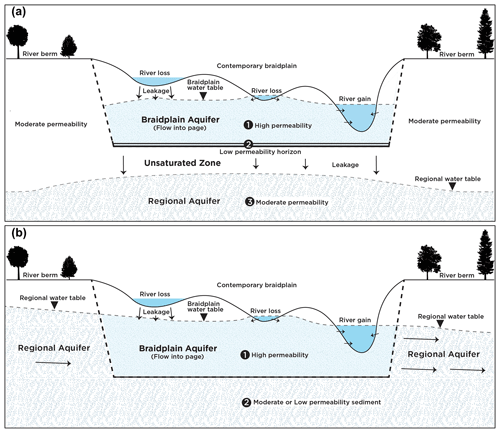
Figure 1Conceptualisation of a braidplain aquifer that is (a) hydraulically disconnected from or transitional with the regional groundwater system and (b) hydraulically connected to the regional groundwater system.
A hydraulically disconnected river system setting shares features in common with intermittent or ephemeral rivers (Shanafield et al., 2021). Infiltration to the regional aquifer is regulated by the vertical resistance of lower-permeability sediments and the hydraulic head in the BPA. If the BPA is fully saturated across the contemporary braidplain, the rate of infiltration to the regional aquifer will be steady because the braidplain has reached a maximum wetted area. Under these conditions, some minor temporal infiltration variability will occur due to changing water levels in the BPA. Under ephemeral conditions, the saturated extent of the BPA decreases longitudinally, and locally laterally, during drying phases in response to extended periods of low river flow. The combined reduction in head and saturated area of wetted braidplain result in considerably less infiltration to the regional aquifer (Di Ciacca et al., 2023).
The second case is a setting in which the river is hydraulically connected to the regional aquifer system (Fig. 1b). A minimum of two lithological units are present: (1) a high-transmissivity BPA and regional aquifer overlying (2) less-permeable sediments that impede vertical flow. The combination of these two factors creates an anisotropy, with preferential flow in the lateral direction. Hydraulically gaining conditions will occur in situations where regional water levels are elevated by low-permeability boundaries (i.e. the presence of bedrock) on one/both sides of the river or at middle to distal positions on alluvial fans where regional groundwater levels are closer to the land surface.
For a hydraulically connected river system (braids and BPA), the hydraulic gradient is no longer vertical, as it is for the hydraulically disconnected scenario, but variable, with the exchange rate governed by the relative hydraulic gradient between the braidplain and regional aquifers. Thus, groundwater inflow to the BPA or discharge to the regional aquifer can occur laterally along both margins of the braidplain as well as vertically through the BPA base. The setting can also be asymmetric, with inflow on one margin and outflow on the other (as shown in Fig. 1b), with the total river system water balance being gaining/losing or having no flow along one margin due to the presence of bedrock.
While the hydraulically connected situation is simpler structurally, relative to the disconnected situation, exchange between the braidplain and regional aquifers is more complex. In this case (Fig. 1b), water exchange is governed by the hydraulic gradient between the two aquifers, the transmissivity of both aquifers and the vertical hydraulic conductivity of the underlying sediments. Once water exits the BPA in a losing reach, it will not return unless it is rerouted back to the river system by a reversal of the hydraulic gradient.
The sedimentological features and groundwater–surface water interaction concepts associated within the contemporary braidplain have been identified and detailed by previous authors (e.g. Huggenberger et al., 1998). Regardless of the nature of the relationship between the braidplain and regional aquifers, the braidplain gravels have a higher transmissivity than both the adjacent and underlying sediments because of repeated reworking of the braidplain gravels during high-flow events. Hyporheic and parafluvial flow occurs within these highly transmissive BPA sediments, subparallel to the river flow direction, with individual braids acting as recharge or discharge boundaries. As such, the local hydraulic gradient and groundwater flux are largely influenced by river bathymetry.
4.1 Catchment descriptions
The conceptualisation presented here is based on field observations of the main losing reaches of three braided rivers in the drier eastern part of Aotearoa / New Zealand (Fig. 2). These are, from north to south, the Ngaruroro (a), Wairau (b) and Waikirikiri (c), the latter of which is also known as the Selwyn. These study areas were selected to take advantage of the potential for hydrological separation afforded by dominantly losing river reaches. The downward hydraulic gradient and the potential hydrological separation between channel, bed gravels and regional aquifer enable the possibility for different structural and hydrological components to be identified. Summary hydrological and geomorphological statistics for the three study sites and their source catchments are shown in Table 1.
Table 1Characteristics of the three study catchments. Mean values are given unless stated otherwise. FRE3 is the annual number of events exceeding 3 times the median flow.
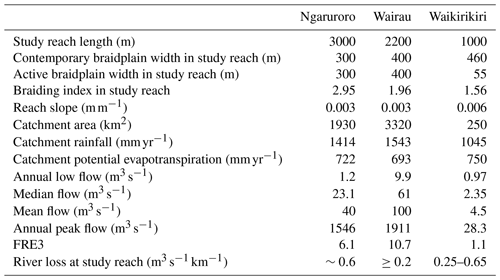
The Ngaruroro River is 164 km long and has its headwaters in the Kaweka, Kaimanawa and Ruahine mountain ranges on the main divide of the North Island. The 3 km long study reach is located at the margin of the Heretaunga Plains between Roy's Hill and Fernhill and is the main recharge source for the Heretaunga alluvial aquifer system (Brown et al., 1999). A long-term flow monitoring site at Fernhill (70 years), situated at the lower end of our study reach, has recorded a mean flow of 40 m3 s−1 and mean annual peak flow of 1546 m3 s−1. The Ngaruroro study reach is in a natural depositional zone within the catchment (i.e. bedload into the reach exceeds that leaving the reach). This reach typically has three braids, with the active braidplain confined between berms that are stabilised by an established buffer of willow tree.
The Wairau River (Wöhling et al., 2018, 2020) is 170 km long and has its headwaters in the alpine Spencer Range on the main divide of the South Island. As such, the Wairau receives considerable source water from rain at higher elevations, producing a mean flow of 100 m3 s−1 and a high mean annual peak flow of 1911 m3 s−1 (63 years of record at SH1). The Wairau River is also “flashy”, with over 10 events exceeding 3 times the median flow annually (FRE3, Booker, 2013). The 2.2 km long study reach is in the middle section of the Wairau Plain, between Jeffries and Giffords roads. This study reach typically has two braids, and the active braidplain is confined between engineered rock revetments and groynes. Bedload flux into and out of this reach is approximately equal (bedload transfer zone).
The Waikirikiri River has its headwaters in the foothills of the Southern Alps and receives considerably less runoff than the other rivers. The 1 km long study reach is situated near Hororata, where the river leaves the foothills and crosses the western margin of the Canterbury Plain. A long-term (58 years) flow monitoring site located upstream of the study reach at Whitecliffs shows a mean annual flow of 4.5 m3 s−1 and a peak annual flow of 28 m3 s−1. The Waikirikiri River is also the least flashy, with an average of only one event each year exceeding a flow of 3 times the median flow. As a result of its lower flow and high transmission losses, the Waikirikiri River is subject to intermittent flow within our study reach (Larned et al., 2008; Di Ciacca et al., 2023). The Waikirikiri study reach is naturally incised between Pleistocene terraces, with no engineered flood controls. The active braidplain, which typically has one to two braids, can freely adjust between these contemporary braidplain margins; however, relatively dense, exotic vegetation covers much of the contemporary braidplain.
All three rivers share a similar bedload lithology dominated by Jurassic greywacke. In the study reaches, the Ngaruroro is bounded to the north by Pliocene siltstone and limestone (Lee et al., 2011), while the Wairau is bounded to the north by schist (Begg and Johnston, 2000). These two rivers lose water southwards, with the river losing reaches being the primary source of recharge for the alluvial aquifers hosted by Holocene gravels. In the Waikirikiri study reach, the river is bounded by Pleistocene glacial outwash gravels (Forsyth et al., 2008). These gravels form the surface expression of a large, stratified aquifer system hosted by a composite of alluvial fans that underlie the Canterbury Plains. The Ngaruroro and Waikirikiri study reaches are both situated close to the apex of the river system's alluvial fan (close to where the rivers emerge from the foothills).
Both the Wairau and Ngaruroro are affected by gravel extraction, which has lowered the mean active braidplain bed elevation in the river recharge reaches by approximately 1 m since the 1980s (Gardner and Sharma, 2016; Measures, 2012). The varying physical environments, flow regimes, bed adjustment trajectories and degree of lateral confinement result in different rates of bed reworking for each river. In particular, the Waikirikiri reworks its bed less frequently than the Ngaruroro and Wairau.
4.2 Field data collection
Investigations in the study reaches involved the collection of a variety of data types (Table 2). Each method and data type has advantages and disadvantages and is scale and process dependent (Gonzalez-Pinzon et al., 2015; Brunner et al., 2017). Stage and temperature recorders were established in the study reaches but were difficult to maintain due to repeated destruction by flood flows and frequent bed movement in the study reaches. Flow rating was conducted at the top of the reach for the Waikirikiri River, and permanent rated flow sites located downstream were used for the Ngaruroro and Wairau. A series of flow-loss gauging surveys were undertaken at multiple transects within all three study reaches across a range of discharges to estimate transmission losses. Due to the difficulty in measuring flow in the larger rivers (and the related large measurement uncertainty), the most comprehensive set of flow-loss surveys was conducted on the Waikirikiri (Di Ciacca et al., 2023).
Table 2Type and number of measurements undertaken in the three study reaches.
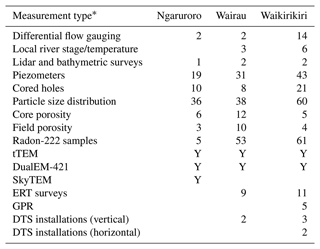
*The abbreviations used in the table are as follows: transient electromagnetic (tTEM), electromagnetic induction (DualEM-421), ground-penetrating radar (GPR), electrical-resistivity tomography (ERT) and passive distributed temperature sensing (DTS). “Y” denotes that a method was used.
Lidar data were captured in dry areas of riverbed using a LiDARUSA Snoopy lidar scanner deployed on either a UAV or backpack. Bathymetry and water surface elevation were mapped using a kayak or a remote-controlled jet boat equipped with a paired real-time kinematic and global positioning system (RTK GPS) and echosounder. In places where the water depth was too shallow for boats, bathymetry and the water’s edge were captured by wading with an RTK GPS. Interpolation or (where necessary) optical-bathymetry techniques were used generate high-resolution bathymetry maps from less-dense echosounder survey data. The dry topography from lidar was stitched together with the bathymetry data to provide a complete digital elevation model (DEM) for each reach at a spatial resolution of 1 m or less with a vertical accuracy of ±0.1 m in dry areas and ±0.2 m in wet areas.
Piezometers were installed at different depths to provide a time series of water levels and temperature as well as to enable sampling for radon-222 analysis. The piezometers were installed with 50 mm diameter PVC pipe. Screens were a 1–2 m length of slotted casing with a geotextile sock and sand pack around the screen as well as cement grout around the overlying casing. A sump was used to collect downward-percolating water in situations where the porewater pressure was below saturation. Drilling methods involved a mix of rotary and sonic drilling, with the former being used to install the piezometer network. Sonic drilling with a Geoprobe 8140LC (76.2 mm diameter core) was conducted to collect cores for detailed logging and sediment analysis (grain size and porosity). The sonic drilling method is not ideal, as it can be difficult to get good core recovery, particularly in coarser or loose near-surface sediments. As a result, the core record is incomplete; however, there is currently no better method available to extract several metres of core from coarse river bed deposits. Because core recovery was variable, data from individual drillholes were fragmented, and sediment analysis was only conducted on the most intact cores (0.1–1.2 m in length), with samples taken for grain size and porosity analysis. Near-surface bed material grain size distribution and porosity were measured using manual excavation sieving combined with a detailed photogrammetry method to calculate excavation volume. Particle size distribution was characterised by fitting Gompertz and Weibull curves (Bayat et al., 2015) to the sieved sediment data, and summary statistics were determined via the method of Folk and Ward (1957).
The base of the BPA was identified in two ways. The first method was by mapping surface morphology, as the deepest areas of pools represent the scouring depth that has occurred during flooding. Surface and bed morphology was captured using remote sensing (photogrammetry, lidar and bathymetry). The second method was via observations using a drill core with good core recovery; here, sediments beneath the BPA are characterised by more cohesion and a finer matrix. To enable data to be compared, contacts between the braidplain gravels and underlying sediments (depositional unconformity) have been expressed as the depth below a detrended surface representing the cross-sectional mean contemporary braidplain surface elevation. This datum was chosen because of the spatially variable and temporally dynamic riverbed levels.
Hydrogeophysical methods were also used to image the subsurface, including passive (DTS) and active (ADTS) distributed temperature sensing (Banks et al., 2022), ground-penetrating radar (GPR), electrical-resistivity tomography (ERT), and transient electromagnetic (tTEM) and electromagnetic induction (DualEM-421). The tracks prepared for tTEM and DualEM surveys are evident in the aerial photos shown in Fig. 3. SkyTEM data were also available for the Ngaruroro area (Rawlinson et al., 2021). Of the hydrogeophysical methods employed, DTS/ADTS and ERT were the most successful methods for delineating sediment structure and saturation associated with the river. The resistivity of braided river water (fluid specific conductance ∼5 mS m−1) and the associated gravel deposits in Aotearoa / New Zealand is very high (400–10 000 Ω m). For this reason, we think there was insufficient resistivity contrast for electromagnetic and ERT methods to reveal distinct subsurface features in most of our surveys. SkyTEM data did provide good definition of the basement contact beneath the Ngaruroro River but did not reveal any clear structural features in the near surface (<10 m). GPR surveys that were trialled at the Waikirikiri site clearly revealed the shallow water table but did not reveal any clear structure beneath the water table due to reflection of the signal.
Samples for radon-222 analysis were collected in 250 mL bottles in the Waikirikiri (Songola, 2022) and Wairau reaches from riffles, at different depths in pools and from purged piezometers. Samples were analysed 21–100 h and 20–24 h after sampling for the Waikirikiri and Wairau respectively. Laboratory analysis for radon activity was conducted with a RAD7 (Durridge, 2020c), RAD H2O (Durridge, 2020b) and active DRYSTIK (Durridge, 2021) in a closed-loop system, with the results adjusted for decay since the time of sampling (WAT250 method). The radon activity and uncertainty values reported here follow the approach of Durejka et al. (2019), with the mean and standard deviation calculated from 5 counting cycles, with duplicate samples pooled (10 cycles total). Additional radon measurements were made in the field using the RAD AQUA method (Durridge, 2020a) to verify the WAT250 method results, and these returned similar values. For this study, we have reported data from the WAT250 method, which has a larger uncertainty associated with the measurements but enables more samples to be collected in a short time frame from remote field sites. To reduce the uncertainty of the WAT250 results, we increased the aeration time to 10 min and increased the analysis duration recommended in the Durridge manual to five cycles of 10 min.
A hydraulically disconnected river–regional aquifer system has been identified from drilling and monitoring data in the Waikirikiri study reach (Banks et al., 2022; Di Ciacca et al., 2023) as well as at the upper part of the Ngaruroro study reach. A hydraulically connected river–regional aquifer system has also been observed in the Wairau study reach as well as in the lower part of the Ngaruroro study reach. Evidence for the proposed conceptualisation is provided below based on data type. Data referred to in the text and figures are shown spatially in Fig. 3. Points where the base of the BPA has been observed in cores or inferred via deep pools are identified.
5.1 Geology and geomorphology
The BPA lateral extent at our three sites is identified geomorphologically as being at the contemporary braidplain margins (orange dashed lines in Fig. 3). The contemporary braidplain margins in our study reaches are either artificially confined by rock armouring/willow planting for flood protection (Wairau and Ngaruroro) or by terrace boundaries (Waikirikiri). These relatively static lateral controls result in the abutment of relatively loose (recently active) braidplain gravels against older, more poorly sorted sediments. In the Wairau and Ngaruroro rivers, the artificial lateral controls are sufficiently narrow that the entire contemporary braidplain is regularly reworked (i.e. the active braidplain margins and contemporary braidplain margins are essentially the same). In the Waikirikiri, the active braidplain is narrower than the contemporary braidplain, and the active braidplain is able to adjust laterally, reworking the contemporary braidplain gravels.
In all three braided rivers, deep pools form at the toe of riffles. In the Wairau and Ngaruroro, these are amplified at the braidplain margins where river flow is reflected by rock armouring or willow tree plantings that limit lateral erosion and enhance bed scour. These pools are zones in which the BPA discharges to the river. Parafluvial seeps are commonly observed at these locations, which have higher radon-222 activities and summer temperatures that are cooler than adjacent river braids. The surface expression of the BPA can be seen in abandoned channels where the braidplain surface topography drops below the braidplain water table and exposes groundwater (static pools or flowing springs). These areas are indicated by dashed red lines in Fig. 3.
The depositional unconformity at the BPA base in recovered cores was observed at 1.7–2.0 m depth beneath the mean bed level of the contemporary braidplain in the Ngaruroro study reach, 4.3–5.0 m depth in the Wairau and 2.1–3.7 m depth in the Waikirikiri. The greater depth in the Wairau River is unsurprising, as this river has the highest flood flows and is tightly confined by engineering works, resulting in greater depths of bed reworking.
At the Waikirikiri site, the contact of the depositional unconformity was seen as a change from grey-brown postglacial sandy gravels to yellow-brown clay-rich glacial outwash gravels (Fig. 4). During drilling and completion of the groundwater monitoring wells, a drop in the water level with depth below the glacial contact was observed. Six cores were drilled just beneath the unconformity at various locations within the contemporary braidplain margins to investigate the saturation status beneath the braidplain gravels. These holes were drilled through the low-permeability contact layer until an apparent increase in permeability was encountered, at which point the water level in the drillhole dropped significantly. Piezometers screened beneath the base of the BPA and above the regional water table showed low and unvarying water levels, indicating unsaturated or variably saturated conditions (Fig. 7). Our explanation for the presence of this variably saturated zone is that the glacial outwash gravels are stratified, and vertical infiltration to the regional aquifer is limited by the lower-permeability horizons of silt and clay. The presence of higher-permeability horizons within the stratified postglacial sequence allows water to move laterally away from the recharge zone at a rate that exceeds vertical infiltration, enabling unsaturated or variably saturated conditions to form.
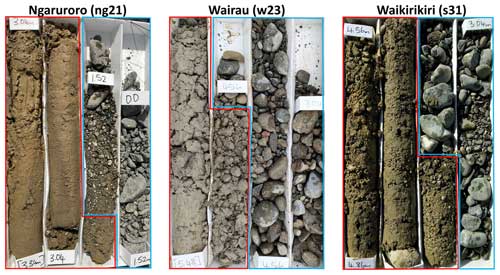
Figure 4Representative core samples across the unconformity at the three study sites (blue – braidplain gravels; red – underlying very poorly sorted and consolidated gravels).
At the Wairau River study site, the base of the BPA is visible as a change from grey-brown sandy-clast-supported gravel to more cohesive and poorly sorted gravel with increasing proportions of silt and clay. Just beneath this unconformity is a more prominent contact with yellow-brown silty clay-bound gravel associated with old outwash fan deposits along the Richmond Range (not shown in Fig. 4 but evident in Fig. 10). Note that some of the Wairau core holes were positioned on the berms (outside of what we are referring to as the contemporary braidplain), where the active braidplain was located prior to river realignment in the 1960s. The river can no longer mobilise sediments on these berms due to rock armouring of the banks leaving a recent remnant braidplain gravel deposit beneath the southern berm. This deposit is both spatially and vertically separate from the contemporary braidplain (the mean bed level of the contemporary braidplain is approximately 2 m below the berms).
At the Ngaruroro study site, the unconformity at the base of the BPA is more gradational, manifesting as an increase in silt and clay content between 1.7 and 2.0 m depth that is visible within the bore logs (Fig. 4) and particle size distribution (Fig. 5). As for the Waikirikiri River, a drop in the water table was observed while drilling beneath this lower-permeability unconformity. The less-distinct base to the BPA in the Ngaruroro study reach is likely because the reach is depositional, so the underlying gravels were deposited relatively recently compared with the other study reaches.
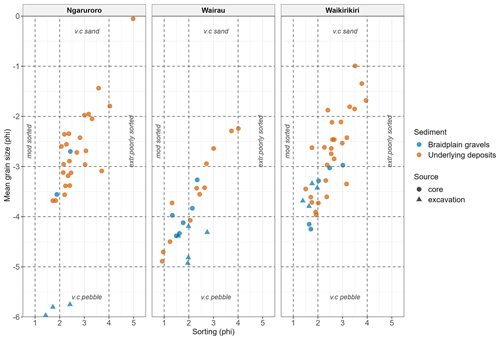
Figure 5Grain size analyses and sorting indices from samples beneath the three study reaches. Dashed lines are breaks for grain size and sorting indices (after Folk and Ward, 1957).
Particle size distribution summary statistics of core samples collected by sonic drilling and bed excavation are shown in Fig. 5, with classifications according to the Folk and Ward (1957) scale. A relationship between sorting and grain size is apparent at each site, with poorer sorting corresponding to an increase in the finer fraction. Shallow braidplain gravels are poorly sorted overall (mean: Ngaruroro 1.98, Wairau 1.90 and Waikirikiri 1.96), whereas the underlying gravels tend to be very poorly sorted (mean: Ngaruroro 2.88, Wairau 2.28 and Waikirikiri 2.60). The grain size of the braidplain gravels is generally coarser (mean: Ngaruroro −4.76, Wairau −4.23 and Waikirikiri −3.55) than the underlying very poorly sorted sediments (mean: Ngaruroro −1.64, Wairau −3.54 and Waikirikiri −2.69).
Core recovery at all sites was typically poor in the contemporary braidplain gravels, which mostly consist of loose gravel and cobbles, with a high proportion of sand but only a trace of silt and clay. Because of this, some of the braidplain gravel samples have been sourced from samples manually excavated from the braidplain subsurface (Fig. 5). These excavated samples exclude the surface armour common in gravel-bed rivers. For the Wairau data set there is no clear separation in the particle size distribution between braidplain and underlying gravels. Wairau sediments are coarser than the other two sites, due to the high energy of this river system. The coarseness of the sediments greatly hampers core recovery, so it is possible that the lack of separation is an artefact of the loss of finer fractions in some samples during drilling.
5.2 Lidar and bathymetry
Lidar and bathymetry surveys were conducted in each study area to understand the spatially varying relationship between the river surface, bed levels and water levels in the braidplain and regional aquifers. Repeat surveys were conducted following significant flood events to capture changes in bed levels. An example of our lidar and bed elevation data for the Wairau River is shown in Fig. 6. These data were captured on 19 February 2020 under relatively low-flow conditions, measured at 13.4–11.5 m3 s−1 (±3 %) at the upstream (left) and downstream (right) margins of Fig. 6 respectively. The river water surface and bed elevation data within the wetted channel are shown in Fig. 6 in relation to a modelled surface of hydraulic head across the river system, represented by piezometric contours (shown in Fig. 6a). This surface was fitted (sum-squared error of ) to 25 water level observations (yellow points in Fig. 6a) located within and outside of the contemporary braidplain by universal kriging with an exponential variogram of anisotropy of 0.9 at 90°, a partial sill of 0.31 m and a range of 670 m. With such a large variogram range, the surface should be considered to be indicative of an averaged hydraulic head across the regional and braidplain aquifers. The kriged surface does reveal an inflection of the piezometric contours across the contemporary braidplain margins, indicating that flow within the BPA is largely controlled by river exchange and preferential flow within the BPA, with flow being approximately sub-parallel to the contemporary braidplain longitudinal orientation.
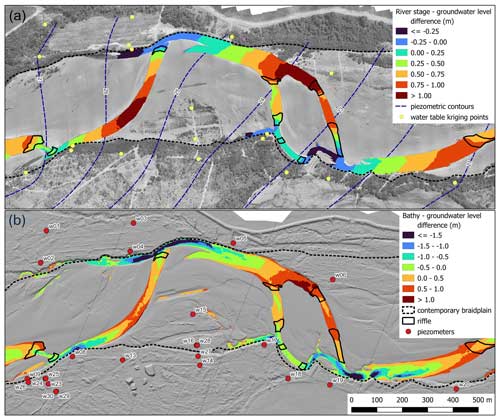
Figure 6Images of the wetted Wairau River channel showing (a) differences between river water surface and a kriged hydraulic head (overlain on aerial imagery) and (b) differences between bathymetry and a kriged hydraulic head (overlain on the DEM). River flow is from left to right.
Figure 6a reveals locations in the river system where the river water surface is higher than the braidplain water table (red and orange zones), indicating that the river is losing flow to the BPA in these areas. Areas of the river that are coloured blue in Fig. 6a represent the surface expression of the braidplain water table in pools. These are locations where the river can potentially gain flow. The black areas denoted as “riffles” are identified from a slope raster derived from the digital elevation model (DEM). Locations where maximum potential river water loss occurs can be identified in most cases as being situated at the upstream margins of high-elevation riffles.
The bathymetry DEM (Fig. 6b) reveals the presence of scouring along the contemporary braidplain margins, which is promoted by excessive river narrowing and rock training banks in the case of the Wairau River. The corollary of this scouring is the relative mounding of gravel in the middle of the contemporary braidplain. The difference between the riverbed level and hydraulic head reveals locations where the riverbed is above the braidplain aquifer, and the river braid has the potential to be losing and hydraulically disconnected at these locations. In most cases these areas also correspond to the upstream margins of high-elevation riffles.
5.3 Water levels and temperature monitoring
Hydraulic heads within the braidplain aquifer are dynamic and fluctuate in response to changes in river stage. Figure 7 shows heads for the Waikirikiri field site, where the base of the BPA is at approximately 207 m elevation and the regional water table is >13 m below the unconformity. The variably saturated zone (porewater pressure below saturation) is at least 10 m thick. For a river system that is hydraulically connected to the regional aquifer, the pressure response outside of the BPA is also dynamic and shows a similar response to the BPA (Fig. 7). For a river system with a hydraulic disconnection, the variably saturated zone attenuates the pressure fluctuations in the regional aquifer (Fig. 7). The relative water level depth and hydraulic response in the regional aquifer can therefore provide a useful test for hydraulic connectivity between the two aquifer systems.
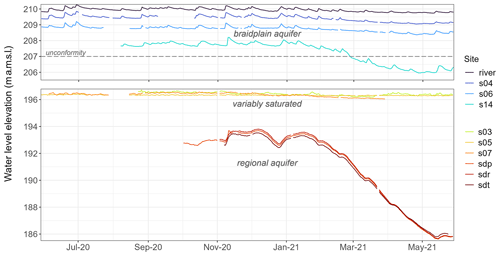
Figure 7Water level time series data for the Waikirikiri River and braidplain and regional aquifers. Note the vertical offset in the two graphs is due to the thick, variably saturated zone beneath the braidplain and regional aquifer. Site locations are shown in Fig. 3.
Data from the Ngaruroro reach show characteristics of a regional aquifer that is both hydraulically connected to the BPA and either disconnected or transitional (Fig. 8). These data have been normalised by the median water level to highlight relative changes in response. The BPA site (ng07) responds similarly to the river stage but has a slower recession rate due to storage in the gravels. The two sites that are screened in the regional aquifer (ng02 and ng04) are both located 150 m adjacent to and downgradient of the contemporary braidplain (see Fig. 3). The upstream site, ng04, has the most rapid recession rate, and its peak response is slightly delayed compared with ng02, which responds similarly to the river channel stage. This suggests that this upstream section of the study reach may be hydraulically transitional or disconnected from the regional aquifer (assuming similar hydraulic properties throughout the regional aquifer). The water levels in ng04 are up to 2.4 m below the BPA base (mean 1.8 m) assuming a BPA thickness of 1.6 m below the mean riverbed level at this site. During flood flow events, the regional aquifer water level at ng04 does rise above the riverbed elevation, indicating that saturated conditions do temporarily occur during flooding.
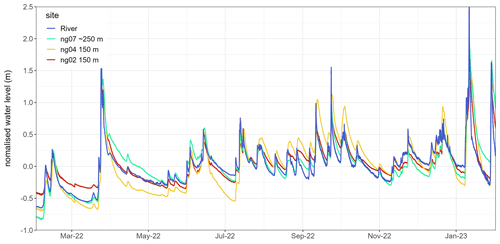
Figure 8Normalised water level time series data for the Ngaruroro River, braidplain (ng07) and regional aquifers (ng02 and ng04). Site locations are shown in Fig. 3. Distances in the legend indicate the downgradient distance from the nearest active river channel.
Figure 9 shows daily average temperatures from representative hydrological settings in each study reach as well as the lateral distance downgradient of the nearest active river channel. For sites located within the BPA, temperature responses are similar to the river channel and are driven by individual flow events (ng07, w09 and s06). However, at large distances from a channel (s25), event-driven responses can be attenuated and the seasonal response can be delayed. Note also that s06 becomes more responsive after a flood event on 30 May 2021 that improved connectivity between the river and groundwater at this piezometer.
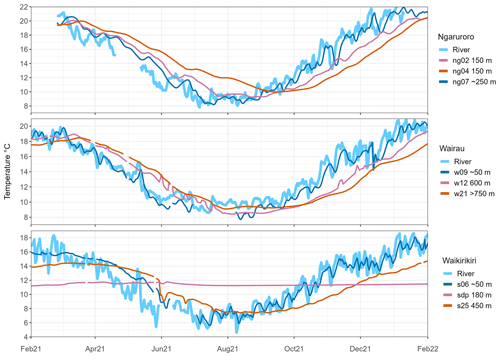
Figure 9Representative temperature time series for the three study sites. Piezometer locations are shown in Fig. 3; sites ng07, w09, s06 and s25 are screened in the BPA.
For sites within the regional aquifer (ng02, ng04, w12, w21 and sdp), the event-driven response is difficult to detect, and the seasonal response depends on the hydraulic relationship between the braidplain and regional aquifers. Sites ng02 and ng04 are equidistant from the Ngaruroro BPA, but ng04 is in a position where the base of the braidplain gravels and the regional water table are approximately 1.8 m apart, potentially with an intervening variably saturated zone, whereas the braidplain and regional aquifers are hydraulically connected at ng02. This difference in hydrologic condition may explain the delayed seasonal response at ng04. In the Waikirikiri system, the variably saturated zone is considerably thicker (about 12 m), which almost completely attenuates the seasonal temperature response in the regional aquifer.
In the Waikirikiri and Ngaruroro river systems, the temperature signals propagate efficiently from river channels to the BPA compared with the regional aquifer. This highlights the distinction between these two aquifers, with the BPA acting as an intermediary in groundwater–surface water exchanges.
5.4 Radon-222 sampling
A summary of the radon-222 results and measurement uncertainties for surface water and groundwater sources in the Wairau and Waikirikiri reaches is shown in Table 3. In the Wairau system, samples from river bed piezometers and riverbed seepages have similar radon activities with ranges of 1724–2849 and 368–2585 Bq m−3 respectively. Accordingly, samples from seepages and river bed piezometers are both considered to represent the braidplain aquifer.
Table 3Measured radon-222 activities and 1 standard deviation uncertainties (Bq m−3) for the Wairau and Waikirikiri study reaches.
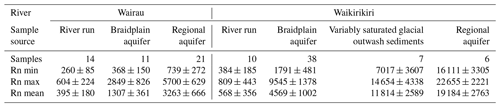
The radon data show distinct groupings, with radon-222 activities increasing from the river channel to the BPA to the regional aquifer. At both sites, radon activities in river run samples were significantly lower than those in BPA samples. In the Wairau study reach, there is a notable overlap in radon activities between the braidplain and regional aquifers, indicating a likely hydraulic connection between these two systems. Conversely, in the Waikirikiri study reach, there is a downward increase in radon activities from the BPA to the variably saturated zone and further into the regional aquifer, with no overlapping values. This suggests a hydraulic disconnection between the BPA and the regional aquifer in the Waikirikiri reach.
The determination of residence times between the river and each aquifer depends on knowing the initial channel condition, the representative secular equilibrium for the host gravel deposit and a well-defined flow path length. Our estimate of the initial river channel condition is 260 Bq m−3 for Wairau and 380 Bq m−3 for Waikirikiri, reflecting the lowest measured river radon-222 activities. A secular equilibrium estimate of 5000 Bq m−3 was derived for Wairau aquifer samples by plotting measured groundwater radon activity against the distance of the piezometer from the river and fitting the ingrowth equation to the data to determine the 21 d equilibrium value. This exercise indicated that the Wairau BPA activities are too low for samples to reach equilibrium. In the absence of sediment-specific data, the Wairau aquifer secular equilibrium was chosen to represent the Wairau BPA equilibrium. The secular equilibrium for the Waikirikiri BPA is estimated to be approximately 8500 Bq m−3 based on the lowest activity observed in porewater samples from piezometer sumps in the variably saturated glacial outwash gravels beneath the braidplain aquifer (7017 Bq m−3) and the highest activity observed in the BPA (9545 Bq m−3). Based on the secular equilibrium values chosen, residence times for our study reach samples are estimated to be in the range of 0.1 to 4.4 d for the Wairau BPA in the study reach and 1 to >12 d for the Waikirikiri BPA. Due to the large uncertainties associated with the WAT250 method, these estimates should be considered for comparative purposes only.
5.5 Geophysics
ERT surveys of the contemporary braidplain in the Wairau and Waikirikiri reaches yielded varying degrees of success. The surveys that returned the most consistent subsurface resistivity response were conducted in the river channel itself, due to the presence of water in the near surface, which improved the connection between electrodes and the underlying resistive substrate. Figure 10 shows dipole–dipole array resistivity profiles across a braid of the Wairau (∼30 m wide) and two braids of the Waikirikiri (∼40 m wide). These profiles reveal the contact between the braidplain aquifer and underlying sediments (the unconformities shown in Fig. 4) at elevations consistent with drillhole coring. For comparison, the elevation of the same unconformity, derived by kriging intercepts of the BPA base within the contemporary braidplain (Fig. 3), is shown in red in Fig. 10. The two profiles are shown at the same spatial and resistivity scale, and an interpretation has been made based on drilling information. Both profiles show a contrast between resistive (∼1000 Ω m) loose sandy gravels that host the BPA and the underlying lower resistivity (<800 Ω m) associated with older, very poorly sorted sediments. The Wairau profile reveals the unconformity at the base of the active braidplain gravels as a less-resistive layer at ∼19.5 m elevation as well as a saturated BPA thickness of ∼3.5 m. On the Waikirikiri profile, the unconformity at the base of the braidplain gravels lies at ∼207.5 m elevation, and the saturated thickness of the BPA is only 2–2.5 m. The underlying very poorly sorted glacial outwash gravels typically show relatively low resistivities (≤600 Ω m) due to the relative abundance of clay-sized sediment, even when not fully saturated.
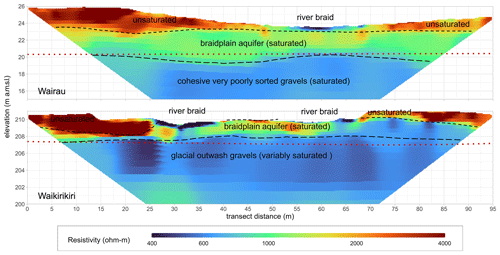
Figure 10Subsurface resistivity collected by electrical-resistivity tomography (ERT) across the Wairau and Waikirikiri river braids, looking downstream. Surfaces interpreted from the resistivity data are shown in black. An interpolated surface for the base of the BPA derived from drilling and bed data is shown in red.
5.6 Passive and active distributed temperature sensing
The hydrogeologic structure in the Wairau study reach has been assessed by monthly DTS and ADTS surveys (Fig. 11) conducted on a vertically installed fibre-optic cable located 20 m from the active braidplain margin (w27 in Fig. 3). While w27 lies just outside of the existing engineered contemporary braidplain, this site does contain remnant braidplain sediments deposited prior to stabilisation of the river margins in the 1960s. The timing of the DTS surveys with respect to river temperature is shown in Fig. 11c. The survey temperature profiles show consistent inflections with depth due to the attenuation of the river recharge temperature response through saturated sediments of contrasting hydraulic conductivity (Fig. 11). These inflections correspond to the depths at which a change in sediment characteristics can be seen in the bore log, manifesting as progressively increased sediment cohesion, poorer sorting, and increasing silt and clay content with depth. In the DTS profile, the older silty, clay-bound and indurated sediments have a large influence on subsurface flow, i.e. a reduction in flow through this material.
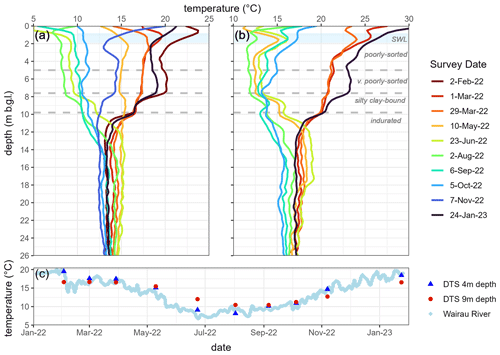
Figure 11Vertical (a) distributed temperature sensing (DTS) and (b) active distributed temperature sensing (ADTS) surveys conducted on the south bank of the Wairau River at w27. The river temperature over the period of the surveys is shown in panel (c) along with temperatures measured by DTS at 4 and 9 m depth. SWL denotes the static water level measurements over the survey period.
A summary of the approximate dimensions and potential maximum groundwater storage volumes of the three braidplain aquifers investigated is shown in Table 4. In all three reaches, the BPA is laterally extensive but very thin.
Table 4Dimensions and maximum storage volumes of braidplain aquifers observed in the three study areas.
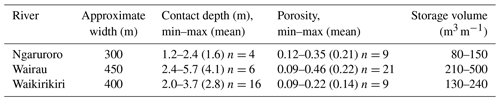
The Wairau River has the greatest BPA storage potential, largely due to its observed saturated thickness of up to 4.1 m. However, the Wairau is also a highly channelised river and has a large bathymetric range, giving its BPA a large potential variability in saturated thickness in response to river channel stage fluctuations. Of significance for water management in the Wairau Plain is that the very poorly sorted gravels are thin beneath the Wairau contemporary braidplain and underlain by buried fans of silty clay-bound and indurated gravels that act as a vertical flow barrier (see Fig. 10). Gravel extraction from the river system has dropped the mean bed level by approximately 1 m within our study reach since the early 1990s (Gardner and Sharma, 2016). This has allowed the river to rework sediment to greater depths, which has thinned and reduced the effective transmissivity of the gravel sequence overlying the buried fan deposits in addition to reducing the hydraulic gradient between the river system and regional aquifer.
The proposed conceptualisation enables a complex braided river system of high complexity to be represented by a few key hydrogeological elements. These are represented in Fig. 1 as a contemporary braidplain, the margins of which mark the lateral extent of the braidplain aquifer (1), an underlying unconformity with more consolidated and more poorly sorted sediments (2), and observations of the hydraulic relationship between the braidplain and regional aquifers (Fig. 1a or b). For braided rivers, this approach integrates existing concepts of an alluvial aquifer and of hydrological hyporheic and parafluvial zones into a single conceptualisation of the contemporary braidplain subsurface. By identifying the base and margins of the BPA and the process that forms it (reworking of bed material), the vertical and lateral extents to which hyporheic and parafluvial exchange occur can be identified by a change in sediment characteristics.
Point-scale features of braided rivers are described well by the existing hydrological framework proposed by Fox and Durnford (2003) and modified by Brunner et al. (2009a, b). Under this framework, individual channels can be considered to be hydrologically connected (gaining or losing), disconnected (losing) or transitional (losing). However, this framework starts to break down for braided rivers at local to sub-catchment scales, as all four of these hydrological states can occur along a single cross-section of the braidplain regardless of whether the river has a net gain or loss. However, at the reach or sub-catchment scale, a braided river can be considered a river system, which can be described by any one of those hydrological states in relation to the regional groundwater system. For example, a river braidplain reach can be hydrologically disconnected and be losing water to groundwater overall, even though individual channels are hydrologically connected and locally gaining flow from BPA groundwater. Thus, the conceptualisation posed by Fox and Durnford (2003) can be applied to different scales within a braided river system, but its application, and therefore interpretation of field measurements, requires knowledge of subsurface structure and saturation.
The difference in sediment characteristics above and below the unconformity at the base of the BPA indicates that the process of BPA formation is controlled by the mobilisation of bed material during flood flows, which loosen and sort the braidplain gravels as well as winnowing the finer fraction. This process of gravel mobility associated with flood events is supported by bathymetric observations of the depth of river channel scouring in deeper pools, which agrees with the depths of the unconformity in core data. In the absence of drill core and particle size distribution data, we suggest that the elevation of pool depths measured soon after a flood event can be used to approximate the base of the BPA. This will only provide a minimum depth of the BPA base, as the river is expected to deposit some sediment in scoured areas during the flow recession. The thickness of a river's BPA is likely to depend on the inter-relationship between several factors, including contemporary braidplain width, sediment characteristics and the balance of sediment supply, the frequency and magnitude of peak flow events, and the use of “hard engineering” to control a river's position. While some of these factors are natural, the factors related to width and depth are influenced by river engineering applied at each river. The Wairau River has the thickest BPA and has the highest hydrological energy of the reaches studied. This river is considered by some river engineers to be excessively narrowed, and a wider contemporary braidplain may result in a dispersion of energy during flood flows as well as subsequent thinning of the BPA. Interestingly, the Ngaruroro River is also subject to high peak flows, although bed-mobilising flows occur less frequently than in the Wairau. It is likely that the Ngaruroro BPA is being thinned by the large volume of gravel extraction occurring within the study reach.
The lidar and bathymetric data gathered from our three study sites indicate that individual river channels locally merge with and diverge from the water table surface of the BPA. Water exchange across the bed is determined by bathymetry and hydraulic properties, where the river can be forced above the water table by gravel lobes or dropped into the water table in locations of scouring. Therefore, areas of relatively still water (pools) are the surface expression of the BPA, with seeps representing locations where the bedform drops below the level of the BPA water table. This explains the occurrence of higher radon-222 activities in pools compared with flowing river channels as well as differences in seasonal water temperature.
For the interpretation of field data, the proposed conceptualisation highlights the importance of identifying and knowing the nature of the relationship between these three potential water sources to interpret observations. For example, to understand the nature and magnitude of river–aquifer interaction by only sampling radon-222 in river channels can be misleading. This is because the radon activity measured in the river depends on both the river setting (run or pool) and the nature of the interaction between the BPA and regional aquifer.
From a modelling perspective, it is questionable if a streambed conductance term is an appropriate physical mechanism for representing braided river–aquifer exchange at the local or catchment scale. The role of bed conductance, if significant, is to regulate exchange between individual river channels and the BPA. Due to the relatively high transmissivity of the bed materials, hyporheic exchange is an integral process of braided river flow, and water can be seen to freely exchange between the surface and the bed. It follows that consideration of water storage in the bed sediments (BPA) with a conductance term to impede flow beneath those sediments would be a more appropriate approach for simulating braided rivers at larger scales than to simulate individual channels with bed conductance.
The BPA concept resolves vague definitions of “groundwater” in groundwater–surface water interactions in braided rivers by considering the river as a whole system with an associated subsurface storage component distinct from the regional groundwater system. This provides specificity to the concept of a “river corridor” (Harvey and Gooseff, 2015), where a river comprises not only the river channels but also the surrounding fluvial deposits, riparian zones and floodplains between which hydrologic exchange occurs.
Braided river systems are spatially and temporally variable, which introduces heterogeneity both within a BPA and the adjacent older sediments. This heterogeneity can manifest as preferential flow paths, which can influence exchange fluxes at a local scale, as evident in the spatial variability in temperature and radon data. While the BPA consists of high-transmissivity sediments and can itself be considered a preferential flow path at the regional scale, the presence of preferential flow within the BPA at the local scale is not captured by the conceptualisation presented here. Therefore, we recommend the application of the BPA concept at the regional scale and to provide a hydrogeological context for local-scale studies. An additional consideration for applying the BPA concept is the volume of reworked material associated with the river. In braided river environments, the volume of gravel associated with the BPA is large (and significantly greater than the wetted channel volume at average flow conditions). However, in some gravel-bed rivers, the volume of sediment mobilised by flooding events could potentially be very thin, and the relevance of these mobile sediments on the exchange between the river and regional groundwater system will depend on the scale of the study.
At the regional scale, the BPA concept is best applied to braided rivers that have stable or actively degrading beds or that have had some form of bank stabilisation, the latter of which is common where flooding is considered a risk to adjacent land. Stabilisation of river margins serves to increase the frequency of gravel remobilisation within the active braidplain and prevent reworking of adjacent bed material that may be part of the historical braidplain. Fine sediment can percolate through the gravels or be deposited on the surface; moreover, if these gravels are not subsequently reworked, this can gradually consolidate and potentially clog the pore spaces, accentuating the difference between contemporary and adjacent historical braidplain sediments. Narrowing the area of braidplain that is reworked can, thus, narrow the BPA. The conceptualisation may be less appropriate or the BPA boundaries may be less distinct in areas where the contemporary braidplain margins are much wider than the active braidplain and reworked over longer time frames. This type of braided river behaviour is typically seen in mountainous areas with low land use pressure (e.g. in the Southern Alps of Aotearoa / New Zealand) but would have occurred on lowland plains prior to the widespread engineering of river margins in Aotearoa / New Zealand during the 1960s. If river channels can adjust laterally over a wider area, it is expected that sediments within the contemporary braidplain will be more heterogeneous, with channels of permeable, recently mobilised gravel intermingled with islands/areas of varying age and permeability. In these cases, the extent of the BPA beyond the active braidplain across the contemporary braidplain may depend on the frequency of contemporary braidplain reworking or the connectivity with the active braidplain. Similarly, it would be difficult to detect the base of the BPA in situations where the river bed is rapidly aggrading.
By investigating the surface and subsurface sediment and saturation in three study reaches, we have developed a conceptualisation of how braided rivers exchange water at the local (reach) and sub-catchment (aquifer) scales. The interaction between the river system and groundwater can be considered to occur between (a) individual river channels and the BPA (hyporheic and parafluvial exchange) or (b) the braidplain and regional aquifers. Central to this conceptualisation is the presence of a braidplain aquifer (BPA), a thin (2–5 m) layer of loose, poorly sorted gravel that is formed via the process of bed mobilisation during flood flows. The base of the BPA can be identified in drill core as an unconformity between poorly sorted, unconsolidated gravels overlying more consolidated, very poorly sorted gravels. Individual river channels can be hydraulically connected, transitional or disconnected from the BPA, depending on the relationship between the braidplain water table and bathymetry. The nature of the hydraulic relationship between the braidplain and regional aquifers can also be hydraulically connected, transitional or disconnected, depending on the relationship between the regional water table and base of the BPA gravels. Approaching the braided river as a (whole) system (river and BPA combined) enables field data to be interpreted within the context of their water source.
From a modelling perspective, the conceptualisation enables rivers to be appropriately represented at a local scale (river–BPA) or a regional scale (BPA/river system–regional aquifer), depending on the modelling objective. A parallel investigation not presented here is focussed on the implementation of the proposed conceptualisation into sub-catchment-scale models to quantify how changes in river morphology and BPA storage influence recharge to the regional groundwater system. A key research gap is to understand the relationship between BPA dimensions, bathymetry and river flow dynamics, and this is a subject for future research.
Data are available upon request from the authors.
SRW wrote the manuscript and prepared the graphs. All of the authors contributed expertise on their specific areas of study to the conceptualisation and reviewed the manuscript. JH and RM collected the aerial photography, lidar, bathymetry and flow data; they also provided geomorphic knowledge. LR analysed the Wairau radon data, and LKM and EWB provided the analysis for all of the radon data. SRW carried out the drilling and lithological analysis. LR undertook the grain size analysis. SRW and EWB collected and analysed the resistivity data. SRW installed the fibre-optic cables and data capture with support from EWB. ADC and TW contributed insights from a modelling perspective. SRW acquired the funding and led the research.
The contact author has declared that none of the authors has any competing interests.
Publisher's note: Copernicus Publications remains neutral with regard to jurisdictional claims made in the text, published maps, institutional affiliations, or any other geographical representation in this paper. While Copernicus Publications makes every effort to include appropriate place names, the final responsibility lies with the authors.
The authors would like to thank the New Zealand Ministry of Business, Innovation and Employment for funding this research through the project “Subsurface processes in braided rivers – hyporheic exchange and leakage to groundwater”. We are also grateful for additional support from Hawkes Bay Regional Council, Marlborough District Council and Environment Canterbury. Moreover, we acknowledge the contribution of the Hydrogeophysics Group at Aarhus University, who carried out the electromagnetic surveys on our rivers, and Christy Songola, who did the radon sampling and analysis at our Selwyn study site. Lastly, we would like to express our gratitude to the reviewers for their valuable comments, which helped us improve the quality of this article.
This research has been supported by the Ministry of Business, Innovation and Employment (grant no. LVLX1901).
This paper was edited by Philippe Ackerer and reviewed by two anonymous referees.
Anderson, E. I.: Modeling groundwater–surface water interactions using the Dupuit approximation, Adv. Water Resour., 28, 315–327, https://doi.org/10.1016/j.advwatres.2004.11.007, 2005.
Banks, E. W., Simmons, C. T., Love, A. J., and Shand, P.: Assessing spatial and temporal connectivity between surface water and groundwater in a regional catchment: Implications for regional scale water quantity and quality, J. Hydrol., 404, 30–49, https://doi.org/10.1016/j.jhydrol.2011.04.017, 2011.
Banks, E. W., Morgan, L. K., Sai Louie, A. J., Dempsey, D., and Wilson, S. R.: Active distributed temperature sensing to assess surface water–groundwater interaction and river loss in braided river systems, J. Hydrol., 615, 128667, https://doi.org/10.1016/j.jhydrol.2022.128667, 2022.
Barthel, R. and Banzhaf, S.: Groundwater and Surface Water Interaction at the Regional-scale – A Review with Focus on Regional Integrated Models, Water Resour. Manag., 30, 1–32, https://doi.org/10.1007/s11269-015-1163-z, 2016.
Bayat, H., Rastgo, M., Mansouri Zadeh, M., and Vereecken, H.: Particle size distribution models, their characteristics and fitting capability, J. Hydrol., 529, 872–889, https://doi.org/10.1016/j.jhydrol.2015.08.067, 2015.
Begg, J. G. and Johnston, M. R.: Geology of the Wellington area. Institute of Geological & Nuclear Sciences 1 : 250 000 geological map 10, GNS Science, Lower Hutt, New Zealand, 1 sheet + 64 pp., ISBN 0478096852, 2000.
Boano, F., Revelli, R., and Ridolfi, L.: Reduction of the hyporheic zone volume due to the stream-aquifer interaction, Geophys. Res. Lett., 35, L09401, https://doi.org/10.1029/2008GL033554, 2008.
Boano, F., Harvey, J. W., Marion, A. Packman, A. I., Revelli, R., Ridolfi, L., and Wörman, A.: Hyporheic flow and transport processes: Mechanisms, models, and biogeochemical implications, Rev. Geophys., 52, 603–679, https://doi.org/10.1002/2012RG000417, 2014.
Booker, D. J.: Spatial and temporal patterns in the frequency of events exceeding three times the median flow (FRE3) across New Zealand, J. Hydrol. NZ, 52, 15–39, 2013.
Boulton, A. J., Findlay, S., Marmonier, P., Stanley, E. H., and Valett, H. M.: The functional significance of the hyporheic zone in streams and rivers, Annu. Rev. Ecol. Syst., 29, 59–81, https://doi.org/10.1146/annurev.ecolsys.29.1.59, 1998.
Bourke, S. A., Cook, P. G., Shanafield, M., Dogramaci, S., and Clark, J. F.: Characterisation of hyporheic exchange in a losing stream using radon-222, J. Hydrol., 519, 94–105, https://doi.org/10.1016/j.jhydrol.2014.06.057, 2014.
Bristow, C. S. and Best, J. L.: Braided rivers: perspectives and problems, in: Braided Rivers, edited by: Best, J. L. and Bristow, C. S., The Geological Society, London, Bath, UK, 1–11, https://doi.org/10.1144/GSL.SP.1993.075.01.01, 1993.
Brower, A., Hoyle, J., Gray, D., Buelow, F., Calkin, A., Fuller, I., Gabrielsson, R., Grove, P., Brierley, G., Sai-Louie, A. J., Rogers, J., Shulmeister, J., Uetz, K., Worthington, S., and Vosloo, R.: New Zealand's braided rivers: The land the law forgot, Earth Surf. Proc. Land., 49, 10–14, https://doi.org/10.1002/esp.5728, 2024.
Brown, L. J., Dravid, P. N., Hudson, N. A., and Taylor, C. B.: Sustainable groundwater resources, Heretaunga Plains, Hawke's Bay, New Zealand, Hydrogeol. J., 7, 440–453, https://doi.org/10.1007/s100400050217, 1999.
Brunner, P. and Simmons, C. T.: Hydrogeosphere: A Fully Integrated, Physically Based Hydrological Model, Groundwater, 50, 170–176, https://doi.org/10.1111/j.1745-6584.2011.00882.x, 2012.
Brunner, P., Cook, P. G., and Simmons, C. T.: Hydrogeologic controls on disconnection between surface water and groundwater, Water Resour. Res., 45, W01422, https://doi.org/10.1029/2008WR006953, 2009a.
Brunner, P., Simmons, C. T., and Cook, P. G.: Spatial and temporal aspects of the transition from connection to disconnection between rivers, lakes and groundwater, J. Hydrol., 376, 159–169, https://doi.org/10.1016/j.jhydrol.2009.07.023, 2009b.
Brunner, P., Therrien, R., Renard, P., Simmons, C. T., and Hendricks Franssen, H.-J.: Advances in understanding river-groundwater interactions, Rev. Geophys., 55, 818–854, https://doi.org/10.1002/2017RG000556, 2017.
Cardenas, M. B. and Zlotnik, V. A.: Three-dimensional model of modern channel bend deposits, Water Resour. Res., 39, 1141, https://doi.org/10.1029/2002WR001383, 2003.
Cartwright, I. and Hofmann, H.: Using radon to understand parafluvial flows and the changing locations of groundwater inflows in the Avon River, southeast Australia, Hydrol. Earth Syst. Sci., 20, 3581–3600, https://doi.org/10.5194/hess-20-3581-2016, 2016.
Coluccio, K. and Morgan, L. K.: A review of methods for measuring groundwater–surface water exchange in braided rivers, Hydrol. Earth Syst. Sci., 23, 4397–4417, https://doi.org/10.5194/hess-23-4397-2019, 2019.
Di Ciacca, A., Leterme, B., Laloy, E., Jacques, D., and Vanderborght, J.: Scale-dependent parameterization of groundwater–surface water interactions in a regional hydrogeological model, J. Hydrol., 576, 494–507, https://doi.org/10.1016/j.jhydrol.2019.06.072, 2019.
Di Ciacca, A., Wilson, S., Kang, J., and Wöhling, T.: Deriving transmission losses in ephemeral rivers using satellite imagery and machine learning, Hydrol. Earth Syst. Sci., 27, 703–722, https://doi.org/10.5194/hess-27-703-2023, 2023.
Durejka, S., Gilfedder, B., and Frei, S.: A method for long-term high resolution 222Radon measurements using a new hydrophobic capillary membrane system, J. Environ. Radioactiv., 208–209, 105980, https://doi.org/10.1016/j.jenvrad.2019.05.012, 2019.
Durridge: Continuous radon in water accessory for the RAD7 user manual, https://durridge.com/documentation/RAD AQUA Manual.pdf (last access: 25 June 2024), 2020a.
Durridge: RAD H2O radon in water accessory for the RAD7 user manual, https://www.geotechenv.com/Manuals/Durridge_Manuals/Durridge_RAD_H2O_Manual.pdf (last access: 25 June 2024), 2020b.
Durridge: RAD7 electronic radon detector user manual, https://durridge.com/documentation/RAD7 Manual.pdf (last access: 25 June 2024), 2020c.
Durridge: DRYSTIK models ADS-3 and ADS-3R active moisture exchanger accessory for the RAD7 user manual, https://durridge.com/documentation/DRYSTIK ADS-3 and ADS-3R Manual.pdf (last access: 25 June 2024), 2021.
Folk R. L. and Ward W. C.: Brazos River bar: a study in the significance of grain size parameters, J. Sediment. Petrol., 27, 3–26, https://doi.org/10.1306/74D70646-2B21-11D7-8648000102C1865D, 1957.
Forsyth, P. J., Barrell, D. J. A., and Jongens, R.: Geology of the Christchurch area. Institute of Geological & Nuclear Sciences 1 : 250 000 geological map 16, GNS Science, Lower Hutt, New Zealand, 1 sheet + 67 pp., ISBN 9780478196498, 2008.
Fox, G. A. and Durnford, D. S.: Unsaturated hyporheic zone flow in stream conjunctive systems, Adv. Water Resour., 26, 989–1000, https://doi.org/10.1016/S0309-1708(03)00087-3, 2003.
Gardner, M. and Sharma, N.: Wairau River Mean Bed Level and Volumetric Analysis: 2010 to 2016, Land River Sea Consulting, Report prepared for Marlborough District Council, 57 pp., 2016.
González-Pinzón, R., Ward, A. S., Hatch, C. E., Wlostowski, A. N., Singha, K., Gooseff, M. N., Haggerty, R., Harvey, J. W., Cirpka, O. A., and Brock, J. T.: A field comparison of multiple techniques to quantify groundwater–surface-water interactions, Freshw. Sci., 34, 139–160, https://doi.org/10.1086/679738, 2015.
Gray, D. P., Hicks, M., and Greenwood, M.: Advances in geomorphology and aquatic ecology of braided rivers, in: Advances in New Zealand freshwater science, edited by: Jellyman, P. G., Davie, T. J. A., Pearson, C. P., and Harding, J. S., New Zealand Freshwater Sciences Society, Christchurch, New Zealand, 357–378, ISBN 9780473376031, 2016.
Harbaugh, A. W.: MODFLOW-2005, the U. S. Geological Survey modular ground-water model – the Ground-Water Flow Process, U. S. Geological Survey Techniques and Methods 6-A16, Reston, VA, USA, 253 pp., https://doi.org/10.3133/tm6A16, 2005.
Harvey, J. and Gooseff, M.: River corridor science: Hydrologic exchange and ecological consequences from bedforms to basins, Water Resour. Res., 51, 6893–6922, https://doi.org/10.1002/2015WR017617, 2015.
Harvey, J. W. and Wagner, B. J.: Quantifying Hydrologic Interactions between Streams and Their Subsurface Hyporheic Zones, in: Streams and ground waters, edited by: Jones, J. B. and Mulholland, P. J., Academic Press, San Diego, CA, USA, 3–44, https://doi.org/10.1016/B978-012389845-6/50002-8, 2000.
Holmes, R. M., Fisher, S. G., and Grimm, N. B.: Parafluvial Nitrogen Dynamics in a Desert Stream Ecosystem, J. N. Am. Benthol. Soc., 13, 468–478, https://doi.org/10.2307/1467844, 1994.
Huber, E. and Huggenberger, P.: Subsurface flow mixing in coarse, braided river deposits, Hydrol. Earth Syst. Sci., 20, 2035–2046, https://doi.org/10.5194/hess-20-2035-2016, 2016.
Huggenberger, P. and Regli, C.: A sedimentological model to characterize braided river deposits for hydrogeological applications, in: Braided Rivers: Process, Deposits, Ecology and Management, Special Publication Number 36 of the International Association of Sedimentologists, edited by: Sambrook Smith, G. H., Best, J. L., Bristow, C. S., and Petts, G. E., Blackwell Publishing, Malden, MA, USA, https://doi.org/10.1002/9781444304374.ch3, 2006.
Huggenberger, P., Hoehn, E., Beschta, R., and Woessner, W.: Abiotic aspects of channels and floodplains in riparian ecology, Freshwater Biol., 40, 407–425, https://doi.org/10.1046/j.1365-2427.1998.00371.x, 1998.
Hupp, C. R. and Osterkamp, W. R.: Riparian vegetation and fluvial geomorphic processes, Geomorphology, 14, 277–295, https://doi.org/10.1016/0169-555X(95)00042-4, 1996.
Kalbus, E., Reinstorf, F., and Schirmer, M.: Measuring methods for groundwater – surface water interactions: a review, Hydrol. Earth Syst. Sci., 10, 873–887, https://doi.org/10.5194/hess-10-873-2006, 2006.
Khambhammettu, P., Renard, P., and Doherty, J.: The traveling pilot point method. A novel approach to parameterize the inverse problem for categorical fields, Adv. Water Resour., 138, 103556, https://doi.org/10.1016/j.advwatres.2020.103556, 2020.
Larned, S. T., Hicks, D. M., Schmidt, J., Davey, A. J. H., Dey, K., Scarsbrook, M., Arscott, D. B., and Woods, R. A.: The Selwyn River of New Zealand: a benchmark system for alluvial plain rivers, River Res. Appl., 24, 1–21, https://doi.org/10.1002/rra.1054, 2008.
Laube, G., Schmidt, C., and Fleckenstein, J. H.: The systematic effect of streambed conductivity heterogeneity on hyporheic flux and residence time, Adv. Water Resour., 122, 60–69, https://doi.org/10.1016/j.advwatres.2018.10.003, 2018.
Lee, J. M, Bland, K. J., Townsend, D. B., and Kamp, P. J. J.: Geology of the Hawkes Bay area, Institute of Geological & Nuclear Sciences 1 : 250 000 geological map 8, GNS Science, Lower Hutt, New Zealand, 1 sheet + 93 pp., ISBN 9780478198225, 2011.
Levy, J., Birck, M. D., Mutiti, S., Kilroy, K. C., Windeler, B., Idris, O., and Allen, L. N.: The impact of storm events on a riverbed system and its hydraulic conductivity at a site of induced infiltration, J. Environ. Manage., 92, 1960–1971, https://doi.org/10.1016/j.jenvman.2011.03.017, 2011.
Measures, R.: Modelling gravel transport, extraction, and bed level change in the Ngaruroro River, National Institute of Water & Atmospheric Research, Client Report CHC2012-121 for Hawkes Bay Regional Council, 55 pp., 2012.
Morel-Seytoux, H. J., Miller, C. D., Mehl, S., and Miracapillo, C.: Achilles' heel of integrated hydrologic models: The stream-aquifer flow exchange, and proposed alternative, J. Hydrol., 564, 900–908, https://doi.org/10.1016/j.jhydrol.2018.07.010, 2018.
Niswonger, R. G. and Prudic, D. E.: Documentation of the Streamflow-Routing (SFR2) Package to include unsaturated flow beneath streams – A modification to SFR1, U. S. Geological Survey Techniques and Methods 6-A13, Reston, VA, USA, 47 pp., https://doi.org/10.3133/tm6A13, 2005.
Pirot, G., Straubhaar, J., and Renard, P.: Simulation of braided river elevation model time series with multiple-point statistics, Geomorphology, 214, 148–156, https://doi.org/10.1016/j.geomorph.2014.01.022, 2014.
Pirot, G., Straubhaar, J., and Renard, P.: A pseudo genetic model of coarse braided-river deposits, Water Resour. Res., 51, 9595–9611, https://doi.org/10.1002/2015WR017078, 2015.
Pirot, G., Huber, E., Irving, J., and Linde, N.: Reduction of conceptual model uncertainty using ground-penetrating radar profiles: Field-demonstration for a braided-river aquifer, J. Hydrol., 571, 254–264, https://doi.org/10.1016/j.jhydrol.2019.01.047, 2019.
Poole, G. C. and Berman, C. H.: An ecological perspective on in-stream temperature: natural heat dynamics and mechanisms of human-caused thermal degradation, Environ. Manage., 27, 787–802, https://doi.org/10.1007/s002670010188, 2001.
Pryshlak, T. T., Sawyer, A. H., Stonedahl, S. H., and Soltanian, M. R.: Multiscale hyporheic exchange through strongly heterogeneous sediments, Water Resour. Res., 51, 9127–9140, https://doi.org/10.1002/2015WR017293, 2015.
Rawlinson, Z. J., Westerhoff, R. S., Foged, N., and Kellett, R. L.: Hawke's Bay 3D Aquifer Mapping Project: Heretaunga Plains SkyTEM data processing and resistivity models, GNS Science consultancy report 2021/93, GNS Science, Wellington, New Zealand, 90 pp., https://data.gns.cri.nz/mapservice/Content/Public/Resistivity/CR2021-93 Heretaunga Plains SkyTEM_FINAL_HBRC.pdf (last access: 25 June 2024), 2021.
Reinfelds, I. and Nanson, G.: Formation of braided river floodplains, Waimakariri River, New Zealand, Sedimentology, 40, 1113–1127, https://doi.org/10.1111/j.1365-3091.1993.tb01382.x, 1993.
Rushton, K.: Representation in regional models of saturated river–aquifer interaction for gaining/losing rivers, J. Hydrol., 334, 262–281, https://doi.org/10.1016/j.jhydrol.2006.10.008, 2007.
Schälchli, U.: The clogging of coarse gravel river beds by fine sediment, Hydrobiologia, 235, 189–197, https://doi.org/10.1007/BF00026211, 1992.
Schilling, O. S., Partington, D. J., Doherty, J., Kipfer, R., Hunkeler, D., and Brunner, P.: Buried paleo-channel detection with a groundwater model, tracer-based observations, and spatially varying, preferred anisotropy pilot point calibration, Geophys. Res. Lett., 49, e2022GL098944, https://doi.org/10.1029/2022GL098944, 2022.
Shanafield, M. and Cook, P. G.: Transmission losses, infiltration and groundwater recharge through ephemeral and intermittent streambeds: A review of applied methods, J. Hydrol., 511, 518–529, https://doi.org/10.1016/j.jhydrol.2014.01.068, 2014.
Shanafield, M., Bourke, S. A., Zimmer, M. A., and Costigan, K. H.: An overview of the hydrology of nonperennial rivers and streams, WIREs Water, 8, e1504, https://doi.org/10.1002/wat2.1504, 2021.
Songola, C.: Characterising Surface Water and Groundwater Interactions in Braided Rivers Using Hydraulics and Environmental Tracers: The Waikirikiri Selwyn River, M.Sc. thesis, Lincoln University, New Zealand, 104 pp., https://hdl.handle.net/10182/15669 (last access: 25 June 2024), 2022.
Sophocleous, M.: Interactions between groundwater and surface water: The state of the science, Hydrogeol. J., 10, 52–67, https://doi.org/10.1007/s10040-001-0170-8, 2002.
Stanford, J. A. and Ward, J. V.: An Ecosystem Perspective of Alluvial Rivers: Connectivity and the Hyporheic Corridor, J. N. Am. Benthol. Soc., 12, 48–60, https://doi.org/10.2307/1467685, 1993.
Steiger, J., Tabacchi, E., Dufour, S., Corenblit, D., and Peiry, J.-L.: Hydrogeomorphic processes affecting riparian habitat within alluvial channel–floodplain river systems: a review for the temperate zone, River Res. Appl., 21, 719–737, https://doi.org/10.1002/rra.879, 2005.
Tang, Q., Schilling, O. S., Kurtz, W., Brunner, P., Vereecken, H., and Hendricks Franssen, H.-J.: Simulating flood induced riverbed transience using unmanned aerial vehicles, physically-based hydrological modelling and the ensemble Kalman filter, Water Resour. Res., 54, 9342–9363, https://doi.org/10.1029/2018WR023067, 2018.
Theel, M., Huggenberger, P., and Zosseder, K.: Assessment of the heterogeneity of hydraulic properties in gravelly outwash plains: a regionally scaled sedimentological analysis in the Munich gravel plain, Germany, Hydrogeol. J., 28, 2657–2674, https://doi.org/10.1007/s10040-020-02205-y, 2020.
Therrien, R., McLaren, R. G., Sudicky, E. A., and Panday, S. M.: HydroGeoSphere: A three-dimensional numerical model describing fully-integrated subsurface and surface flow and solute transport, Groundwater Simulations Group, University of Waterloo, Waterloo, ON, 456 pp., https://www.ggl.ulaval.ca/fileadmin/ggl/documents/rtherrien/hydrogeosphere.pdf (last access: 25 June 2024), 2010.
Valett, H. M, Morrice, J. A., Dahm, C. N., and Campana, M. E.: Parent lithology, surface–groundwater exchange, and nitrate retention in headwater streams, Limnol. Oceanogr., 41, 333–345, https://doi.org/10.4319/lo.1996.41.2.0333, 1996.
Warburton, J.: Active braidplain width, bed load transport and channel morphology in a model braided river, J. Hydrol. NZ, 35, 259–285, 1996.
Ward, A. S.: The evolution and state of interdisciplinary hyporheic research, WIREs Water, 3, 83–103, https://doi.org/10.1002/wat2.1120, 2015.
Ward, A. S. and Packman, A. I.: Advancing our predictive understanding of river corridor exchange, WIREs Water, 6, e1327, https://doi.org/10.1002/wat2.1327, 2019.
White, D. S.: Perspectives on defining and delineating hyporheic zones, J. N. Am. Benthol. Soc., 12, 61–69, https://doi.org/10.2307/1467686, 1993.
White, P. A., Kovacova, E., Zemansky, G., Jebbour, N., and Moreau-Fournier, M.: Groundwater-surface water interaction in the Waimakariri River, New Zealand, and groundwater outflow from the river bed, J. Hydrol. NZ, 51, 1–23, 2012.
Wöhling, T., Gosses, M. J., Wilson, S. R., and Davidson, P.: Quantifying River-Groundwater Interactions of New Zealand's Gravel-Bed Rivers: The Wairau Plain, Groundwater, 56, 647–666, https://doi.org/10.1111/gwat.12625, 2018.
Wöhling, T., Wilson, S., Wadsworth, V., and Davidson, P.: Detecting the cause of change using uncertain data: Natural and anthropogenic factors contributing to declining groundwater levels and flows of the Wairau Plain aquifer, New Zealand, J. Hydrol., 31, 100715, https://doi.org/10.1016/j.ejrh.2020.100715, 2020.
Wu, F.-C. and Huang, H.-T.: Hydraulic Resistance Induced by Deposition of Sediment in Porous Medium, J. Hydraul. Eng., 126, 547–551, https://doi.org/10.1061/(ASCE)0733-9429(2000)126:7(547), 2000.
Wu, G. D., Shu, L. C., Lu, C. P., Chen, X. H., Zhang, X. Appiah-Adjei, E. K., and Zhu, J. S.: Variations of streambed vertical hydraulic conductivity before and after a flood season, Hydrogeol. J., 23, 1603–1615, https://doi.org/10.1007/s10040-015-1275-9, 2015.
Zhou, Y., Ritzi, R. W., Soltanian, M. R., and Dominic, D. F.: The influence of streambed heterogeneity on Hyporheic flow in gravelly Rivers, Groundwater, 52, 206–216, https://doi.org/10.1111/gwat.12048, 2014.
- Abstract
- Introduction
- Review of existing concepts
- Proposed conceptualisation
- Locations and methods for concept validation
- Field observations
- Characteristics of the identified braidplain aquifers
- Discussion
- Conclusions
- Data availability
- Author contributions
- Competing interests
- Disclaimer
- Acknowledgements
- Financial support
- Review statement
- References
- Abstract
- Introduction
- Review of existing concepts
- Proposed conceptualisation
- Locations and methods for concept validation
- Field observations
- Characteristics of the identified braidplain aquifers
- Discussion
- Conclusions
- Data availability
- Author contributions
- Competing interests
- Disclaimer
- Acknowledgements
- Financial support
- Review statement
- References