the Creative Commons Attribution 4.0 License.
the Creative Commons Attribution 4.0 License.
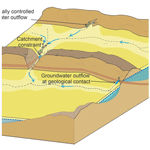
A hydrological framework for persistent pools along non-perennial rivers
Margaret Shanafield
Paul Hedley
Sarah Chapman
Shawan Dogramaci
Persistent surface water pools along non-perennial rivers represent an important water resource for plants, animals, and humans. While ecological studies of these features are not uncommon, these are rarely accompanied by a rigorous examination of the hydrological and hydrogeological characteristics that create or support persistent river pools. Here we present an overarching framework for understanding the hydrology of persistent pools. Perched surface water, alluvial water throughflow, and groundwater discharge are the key hydraulic mechanisms that control the persistence of pools along river channels. Groundwater discharge can be further categorized into that controlled by a geological contact or barrier and discharge controlled by topography. Emphasis is put on clearly defining throughflow of alluvial water and the different drivers of groundwater discharge. The suite of regional-scale and pool-scale diagnostic tools available for elucidating these hydraulic mechanisms are summarized and critiqued. Water fluxes to pools supported by throughflow alluvial and groundwater discharge can vary spatially and temporally, and quantitatively resolving pool water balance components is commonly non-trivial. This framework allows for the evaluation of the susceptibility of persistent pools along river channels to changes in climate or groundwater withdrawals. Finally, we demonstrate the application of this framework using a suite of the available tools to conduct a regional and pool-scale assessment of the hydrology of persistent river pools in the Hamersley Basin of north-western Australia.
- Article
(22825 KB) - Full-text XML
- BibTeX
- EndNote
Permanent or almost permanent water features along non-perennial rivers (hereafter referred to as “persistent pools”) represent an important water resource for plants, animals, and humans. These persistent pools typically hold residual water from periodic surface flows, but also may receive input from underlying aquifers, and have alternately been termed pools (Bogan and Lytle, 2011; Jaeger and Olden, 2011; John, 1964), springs (Cushing and Wolf, 1984), water holes (Arthington et al., 2005; Bunn et al., 2006; Davis et al., 2002; Hamilton et al., 2005; Knighton and Nanson, 2000; Rayner et al., 2009), and wetlands (Ashley et al., 2002). Non-perennial streams are globally distributed across all climate types (Shanafield et al., 2021; Messager et al., 2021). The occurrence of persistent pools along non-perennial streams has been well documented (Bonada et al., 2020), particularly in the arid south-west of the US (Bogan and Lytle, 2011) and across Australia (Arthington et al., 2005; Bunn et al., 2006; Davis et al., 2002).
Several studies have confirmed that persistent river pools support a highly diverse community of flora and fauna (Shepard, 1993; Bonada et al., 2020) and can vary significantly in water quality (Stanley et al., 1997). Persistent pools are also often of cultural significance (Finn and Jackson, 2011; Yu, 2000), providing key connectivity across landscapes for biota (Sheldon et al., 2010; Goodrich et al., 2018) and early hominid migration (Cuthbert et al., 2017). Paradoxically, the unique ecosystems they support are also sensitive to changing climate and human activities (Bunn et al., 2006; Jaeger and Olden, 2011). Persistent pools may dry out naturally after successive dry years (Shanafield et al., 2021), and recent studies have shown that persistent pools are also changing over time in response to alterations in climate and sediment transport (Pearson et al., 2020; Bishop-Taylor et al., 2017). However, their hydrology is typically poorly understood, and the treatment of the hydrology of persistent river pools in published literature to date has been largely descriptive, vague, or tangential to the main theme of the paper (Thoms and Sheldon, 2000). As a result, effective water resource management is limited by a lack of understanding of the mechanisms and water sources that support these persistent pools.
By far, the published literature on persistent pools focuses on the ecological processes and patterns. They have received attention for the role they play as a seasonal refuge (Goodrich et al., 2018) and with regards to connectivity between riparian ecosystems (Godsey and Kirchner, 2014). For example, they have been shown to host unique fish assemblages (Arthington et al., 2005; Labbe and Fausch, 2000), macroinvertebrate communities (Bogan and Lytle, 2011), and play a vital role in primary productivity (Cushing and Wolf, 1984). Recently, it was shown that the structure, but not composition, of persistent pools mirrors that of perennial rivers (Kelso and Entrekin, 2018). However, rarely are these ecological studies accompanied by a rigorous examination of the hydrological and hydrogeological characteristics that provide a setting for these ecologic communities. Although there are isolated studies that examine the composition of water and propose sources within specific pools (Hamilton et al., 2005; Fellman et al., 2011), more frequently they simply describe the seasonal persistence of flow and basic hydrologic parameters (typically temperature and salinity, sometimes also oxygen).
From a hydrogeological perspective, classification of persistent pools, and springs in general, dates back to the early 20th century, when geological drivers such as faults and interfaces between bedrock and the overlying alluvial sediments were first discussed in relation to springs (Bryan, 1919; Meinzer, 1927). Subsequently, a diverse, modern toolbox of hydrologic and hydrogeologic field and analysis methods to analyse water source, age, and composition has evolved. Yet contemporary work on springs (Alfaro and Wallace, 1994; Kresic, 2010) and hydrogeology textbooks (e.g. Fetter, 2001; Poeter et al., 2020) are still based primarily on these early classifications. More recent classifications, moreover, are either descriptive or focus on the context (karst vs. desert) or observable spring water quality (Springer and Stevens, 2009; Shepard, 1993; Alfaro and Wallace, 1994) and are not readily applied to understand the hydrology of persistent river pools (not all persistent pools are springs). There has also been a robust body of literature developed around surface water–groundwater interaction of the past 20 years (e.g. Stonedahl et al., 2010; Winter et al., 1998), some of which informs our understanding of persistent river pools, but has not yet been explicitly applied in this context. Similarly, our understanding of the hydrology of non-perennial streams and their links to groundwater systems continues to expand (Costigan et al., 2015; Gutiérrez-Jurado et al., 2019; Blackburn et al., 2021; Bourke et al., 2021). The existence of rain-fed freshwater rock pools that are not connected to the groundwater system has also been documented in the context of understanding their ecology (Jocque et al., 2010), but the discussion of their hydrology is limited. Thus, while many of the hydrologic concepts relevant to persistent river pools can be found in existing literature, a comprehensive hydrologic framework is lacking (Costigan et al., 2016; Leibowitz et al., 2018). Such a framework should incorporate the relevant elements of literature on groundwater springs and surface–groundwater interaction, along with the modern suite of diagnostic tools, to provide a robust platform for understanding the hydraulic mechanisms that support persistent river pools.
The aim of this paper is to consolidate the hydrologic processes and observational diagnostic tools within existing literature into a cohesive framework to support the characterization of the hydrology of persistent pools along non-perennial rivers. To this end, we (i) identify the range of hydraulic mechanisms supporting river pool persistence during periods of no-flow and show how these mechanisms can manifest in the landscape, (ii) discuss the resulting susceptibility of pools to changing climate or groundwater withdrawals, and (iii) present and critique field-based observational tools available for identifying these hydraulic mechanisms. The application of this framework is demonstrated as a regional-scale assessment and three pool-scale studies from the Hamersley Basin of north-western Australia.
Here we propose a framework for classifying the key hydraulic mechanisms that support the persistence of pools along non-perennial rivers (summarized in Table 1). Geologically, we start by considering the general case of a non-perennial river along an alluvial channel (inundated and/or flowing during contemporary flood events) within valley-fill sediments deposited over bedrock, where tributaries flow either across the bedrock or the valley-fill sediments (Sect. 2.1 and 2.2). We then move onto a discussion of the ways in which geological structures and outcrops can underpin the persistence of river pools by facilitating the outflow of regional groundwater (Sect. 2.3). The range of geological settings for non-perennial streams is vast (Shanafield et al., 2021); we have endeavoured to provide sufficient general guidance so that the principles can be applied to specific river systems as required. Hydrologically, we only consider the water balance of residual river pools after surface flows have ceased. Any water that has infiltrated to the subsurface saturated zone (which may be a perched aquifer) is considered to be groundwater, irrespective of the residence time of that water in the subsurface. This groundwater may be alluvial groundwater stored within the alluvium beneath and adjacent to the contemporary river, or regional groundwater stored within regional aquifers. The conceptual diagrams presented in this section are intended as generalized diagrams to represent key hydrological features; they do not represent specific locations and are not drawn to scale.
Table 1Summary of hydrological framework for persistent pools.
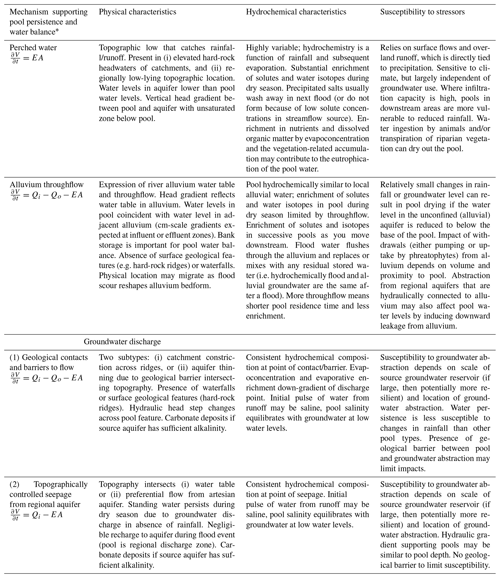
* Water balance of residual pool when disconnected from surface water flows in the absence of rainfall and if only one mechanism is operating. V is the volume of water in the pool (L3), t is time (T), Qi is the water flux from the subsurface into the pool (L3 T−1), Qo is the water flux out of the pool into the subsurface (L3 T−1), E is the evapotranspiration rate (L T−1), and A is the surface area of the pool (L2).
2.1 Perched surface water
Perched surface water can persist in topographic lows that retain rainfall and runoff during the dry season but are disconnected from the groundwater system (Fig. 1). This can be the case if the pool directly overlies impermeable bedrock, or if there is a low-permeability layer between the pool and the water table (Brunner et al., 2009). The presence of this low-permeability layer is essential to maintain a surface water body that is disconnected from the groundwater system. In the absence of a low-permeability layer, the surface water will slowly infiltrate into the subsurface (Shanafield et al., 2021). This low-permeability layer typically consists of clay, cemented sediments (e.g. calcrete), or bedrock (Melly et al., 2017; Joque et al., 2010). The persistence of water in these pools will depend on (a) shading from direct sunlight, and/or (b) sufficient water volume so that it is not completely depleted by evapotranspiration during the dry season (which will be largely a function of pool depth). Given the lack of a water replenishment mechanism during the dry season, these pools may persist for some, but not all, of the dry season.
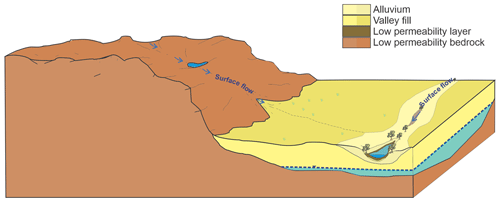
Figure 1Schematic illustration of perched pools where rainfall–runoff collects in a depression that has morphology that limits evaporation, and/or low permeability lithology beneath the pool that limits infiltration, allowing water to be retained for an extended duration.
The occurrence and biological significance of such perched pools has been described particularly for rivers in inland Australia, where contribution of groundwater has been ruled out on the basis of pool hydrochemistry (e.g. Bunn et al.., 2006; Fellman et al., 2011). It should be noted that definitive characterization of perched surface water (i.e. disconnected from the groundwater system) requires the measurement of a vertical hydraulic gradient between the water level in the pool and local groundwater, as well as identification of a low-permeability layer at the base of the surface water (Brunner et al., 2009). Although the ecological significance of perched instream pools is documented within the literature (Boulton et al., 2003; Arthington et al., 2005; Bonada et al., 2020), there is typically no detailed analysis of the hydrology, and sampling is synoptic, so the mechanism of persistence is unclear.
2.2 Throughflow of alluvial groundwater
During rainfall events, increases in water levels in rivers result in water storage and flow within the unconsolidated alluvial sediments in the beds and banks of stream channels (Cranswick and Cook, 2015). As the streamflow recedes after a flood, continuous surface flow ceases, resulting in isolated pools along the river channel. Some vertical thickness of the alluvial sediments that line the stream channel will remain saturated with water beyond the period of surface water flow; this water is hereafter referred to as alluvial groundwater. Although the subsurface residence times of this alluvial water may be on the order of months to years (Doble et al., 2012), this water can be accurately described as groundwater. As such, alluvial water can be considered as a groundwater storage (Leibowitz and Brooks, 2008). Persistent river pools can be expressions of this water within streambed sediments (Fig. 2); this source of water, limited to the floodplain, distinguishes the throughflow mechanism from regional groundwater discharge.
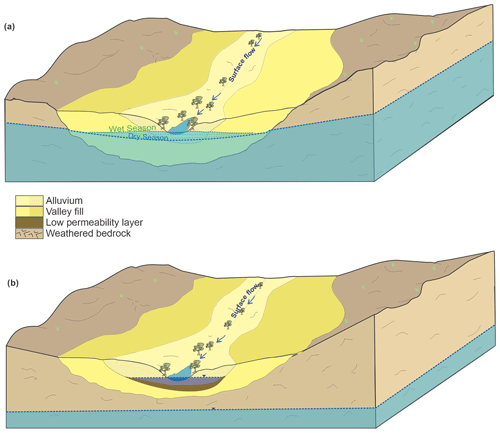
Figure 2Schematic illustration of pools that are maintained by throughflow from the adjacent alluvial sediments. The water in these alluvial sediments can be either (a) connected to the unconfined aquifer, or (b) form a perched aquifer if the water is stored over a low permeability layer.
Alluvial groundwater can be either perched above, or connected to, the regional unconfined aquifer depending on the depth of the regional water table and the presence of a low-permeability layer to enable perching (Villeneuve et al., 2015; Rhodes et al., 2017). Once within the alluvial sediments, this water can subsequently (1) flow laterally through the alluvial sediments towards the bottom of the catchment, (2) be lost to the atmosphere through evapotranspiration, or (3) migrate vertically downward into lower geological layers (Brooks and Hayashi, 2002; Shanafield et al., 2021; Leibowitz and Brooks, 2008). The recession of groundwater levels in the alluvium will therefore depend on the amount of water stored, hydraulic gradients and the permeability of the sediments (which control lateral groundwater flow), hydraulic connections to underlying aquifers (facilitating vertical leakage), and evapotranspiration (Brooks and Hayashi, 2002; Doble et al., 2012; McCallum and Shanafield, 2016). The water level and hydraulic gradients adjacent to persistent pools supported by alluvial throughflow can therefore change seasonally in response to alluvial recharge by rainfall events and subsequent depletion of water stored in the sediments through water flowing into the pool, evapotranspiration. and subsurface groundwater flow (Käser et al., 2009; McCallum and Shanafield, 2016).
The storage and movement of water within alluvial sediments beneath and adjacent to streams has been described extensively in literature on hyporheic exchange (e.g. Stonedahl, 2010) with water fluxes across temporal (days to weeks) and spatial scales (centimetres to tens of metres). From a hydrological perspective, the key feature of the hyporheic zone, and hyporheic exchange fluxes, is that it is a zone of mixing between surface water and shallow groundwater. The scales and mechanisms of hydraulic fluxes (water movement in and out of the streambed) are determined by streamflow and channel morphology, which control hydraulic gradients (Stonedahl et al., 2010; Bourke et al., 2014a). Thus, when the stream is not flowing, the in-and-out hyporheic exchange fluxes that are driven by streamflow are not operating. However, there can still be exchange of water between surface pools and alluvial water driven by hydraulic gradients between the pool and alluvial water.
Some authors have considered this exchange through the lens of the hyporheic zone (Käser et al., 2009; Rau et al., 2017; DelVecchia et al., 2022). However, the hydraulic gradients controlling water fluxes between pool and alluvium in a stream that is not flowing are not related to changes in stream elevation along pool–riffle sequences; rather, they are controlled by hydraulic gradient between the pool and water within the alluvium. While alluvial water that is perched above, and not connected to, the regional aquifer does not fit the dominant conceptualization of hyporheic exchange, the physical process that links streambed elevation changes to flow paths beneath pool–riffle sequences in flowing streams can be relevant to persistent instream pools, regardless of connection status (DelVecchia et al., 2022). When considering the hydraulic gradient between the pool and the water beside it (as opposed to beneath it), this can be described as parafluvial flow (Bourke et al., 2014a). The transient process of alluvial groundwater recharge and subsequent draining of stored water is also analogous to “bank storage” adjacent to flowing streams.
An alternative lens through which to consider the exchange of water between persistent river pools and alluvial groundwater is provided by throughflow lakes, a well-established concept in literature on surface water–groundwater interaction (Winter et al., 1998). There is a comprehensive body of literature on the dynamics of throughflow lakes (Turner and Townley, 2006, Zlotnik et al., 2009; Ong et al., 2010; Befus et al., 2012). Based on this conceptualization, alluvial water will flow into the pools from the subsurface across the upstream portion, and out of the pool, into the subsurface across the downstream portion (Townley and Trefry, 2000; Zlotnik et al., 2009). This conceptualization has the advantage of concisely describing theoretical hydraulic gradients and water exchange between the pool and the entirety of the surrounding alluvial groundwater, while also accounting for the modification of the hydraulic head distribution of alluvial groundwater caused by the pool itself. The water level in pools supported by this alluvial groundwater is effectively a window into the water table within the streambed sediments (Townley and Trefry, 2000). The rate of inflow to (and outflow from) the pool is dependent on the hydraulic conductivity of the sediments (Käser et al., 2009), and the balance of inflow and outflow controls the depth and residence time of water in the pools (Cardenas and Wilson, 2007). The duration of persistence of the pool will also depend on the storage capacity of the alluvial sediments that support it; these pools may dry seasonally (Rau et al., 2017) or persist throughout the dry season if the water level in the alluvial sediments remains above the elevation of the pool.
2.3 Regional groundwater discharge
Similar to springs, rivers can be discharge points for regional groundwater, and this discharge can support the persistence of instream pools during periods without surface flow. Groundwater discharge through springs has been articulated into a range of detailed and complex categories, which are not consistent within the literature (Bryan, 1919; Springer and Stevens, 2009; Kresic and Stevanovic, 2010). These existing spring classifications are based on geological mechanism, hydrochemical properties, landscape setting, or a combination of all three, leading to broad categories such as thermal or artesian, as well as nuanced distinctions based on detailed geological structures (Alfaro and Wallace, 1994). For the purposes of understanding persistent river pools, this array of categories is both overly complex and incomplete, from a hydraulic point of view. For example, Springer (2009) presents a classification of springs based on their “sphere of influence”, which is the setting into which the groundwater flows. A “limnocrene spring” is simply any groundwater that discharges to a pool, as distinct from say a “cave spring”, which emerges into a cave. On this basis, one might consider all persistent pools that are not perched as limnocrene springs. However, the schema also articulates `helocrene springs” which are associated with wetlands and “rheocrene springs” that emerge into stream channels. These also seem to be potentially fitting labels for persistent river pools, which does one choose? And what would it matter for water resource management and the conservation of pool ecosystems if you chose one category over the other?
We suggest two broad categories can encompass the range of hydraulic mechanisms supporting persistent pools in intermittent stream channels; geological features (i.e. lithologic contacts and barriers to flow) and topographic lows. This distinction is valuable because it facilitates an understanding of the source of groundwater discharge (shallow, near-water table vs. deeper groundwater) and the size of the reservoir supporting the pool, both of which contribute to the susceptibility of pool persistence to groundwater pumping. This distinction can also be useful for identifying the dominant hydrogeological control on the influx of regional groundwater to the pool; in hard-rock settings with geological contacts and barriers, the influx may be limited by the effective hydraulic conductivity, whereas in a topographic low, the influx will be controlled by hydraulic head gradient between the pool and the groundwater source (see case studies below).
2.3.1 Geological contacts and barriers to flow
Geological contacts are well established as potential drivers of groundwater discharge through springs (Bryan, 1919; Meinzer, 1927). For example, contact springs occur where groundwater discharges over a low-permeability layer commonly associated with springs along the side of a hill or mountain (Kresic and Stevanovic, 2010; Bryan, 1919). Similarly, pool persistence can be supported by groundwater discharge into a stream channel over a low-permeability geological layer caused by the reduced vertical span of the aquifer (Fig. 3a); where this vertical span reduces to zero is known colloquially as the aquifer “pinching out”. This mechanism has been identified as driving regional groundwater discharge to streams (Gardener et al., 2011), but to our knowledge has not yet been explicitly discussed in the context of persistent river pools.
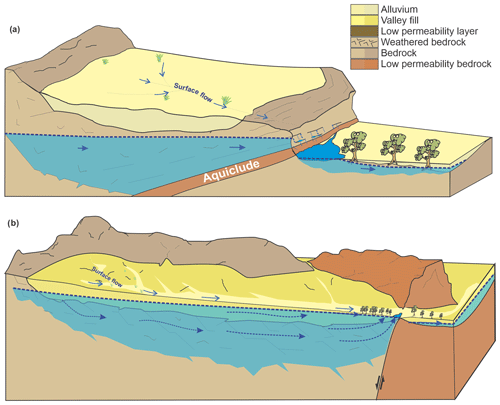
Figure 3Schematic illustration of a groundwater discharge pools where surface water persistence is driven by geological barriers that (a) cause a regional aquifer to pinch out vertically or (b) form a lateral constraint on the catchment and underlying regional aquifer.
Outflow of groundwater where a catchment is constrained by hard-rock ridges that constrict groundwater flow (by reducing the lateral span of surface flow and the aquifer) can also support the persistence of surface water pools (Fig. 3b). Although the importance of catchment constriction has been identified by practitioners (e.g. Queensland Government, 2015), to our knowledge the discharge of groundwater caused by catchment constriction as a mechanism for surface water generation has not previously been described in published literature (springs or otherwise).
2.3.2 Topographically controlled seepage from regional aquifers
Pool persistence can be sustained by groundwater seepage from regional aquifers in the absence of geological barriers or contacts if there is a topographic low that intersects the regional water table (Fig. 4). This mechanism will generally occur where differential erosion causes a difference in topography, which is equivalent to depression springs (Kresic and Stevanovic, 2010; Bryan, 1919) and analogous to the lakes that form in pit voids left after mining ceases (McJannet et al., 2017). For example, pools likely supported by this mechanism have been identified within the Adelaide region of South Australia where erosion within a syncline has exposed bedrock, facilitating groundwater discharge (Lamontagne et al., 2021). Within the humid landscape of south-eastern USA, Deemy and Rasmussen (2017) also describe a vast number of pools along intermittent streams. These pools, which are seasonally connected by surface flows during the wet season, are expressions of the karst groundwater networks that underlie them, and may be considered special cases of topographically-controlled groundwater discharge pools. Topographic depressions that fill seasonally with water, known as “sloughs” on the North American prairie, operate similarly hydraulically (seasonal snowmelt inputs, evaporation induces groundwater inflow), but these sloughs are not within river channels and commonly reside within low-permeability glacial clays so that they are supported by the local-scale groundwater system (Van der Kamp and Hayashi, 2009). Even some Arctic lakes, formed in shallow topographic depressions, receiving groundwater input and seasonally situated within a stream of snowmelt runoff (Gibson, 2002) can be considered as pools supported by topographically-controlled groundwater discharge.
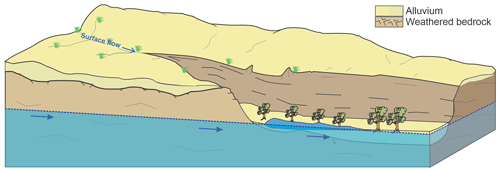
Figure 4Schematic illustration of a pool receiving topographically-controlled groundwater outflow from a regional aquifer.
Pools may also be sustained by topographically-controlled seepage from confined aquifers if there is a fault or fissure that acts as a conduit to groundwater flow (different to Fig. 3a because there is no geological transition to sustain a hydraulic gradient across the pool). Topographically-controlled discharge from a confined aquifer is analogous to artesian mound springs like those found in the Great Artesian Basin of central Australia (Ponder, 1986), but these do not reside within non-perennial streams. Groundwater discharge along fractures or faults has been identified as an important mechanism for groundwater discharge to the Fitzroy River in northern Australia (Harrington et al., 2013), but the significance of this regional groundwater discharge to individual persistent pools is not yet known.
Robust water resource management in semi-arid regions requires an understanding of the ways in which human activities or shifting climates can alter water balances and/or the duration of pool water persistence (Caldwell et al., 2020; Huang et al., 2020). In the absence of published literature quantifying the susceptibility of persistent pools, we present general guidance on the susceptibility of pools to changes in rainfall and groundwater withdrawals based on hydrologic principles (Table 1).
Intuitively, the size of the reservoir (surface catchment or groundwater storage) that supplies water to the pool should be a key factor in determining the susceptibility of persistent pools to changing hydrological regimes. However, the patchiness of rainfall and substantial transmission losses typical of semi-arid zone intermittent river catchments (Shanafield and Cook, 2014) means that for pools reliant on surface catchments (perched or supported by alluvial throughflow), catchment size alone is unlikely to be a robust predictor of resilience. As has been demonstrated for arid zone wetlands in Australia (Roshier et al., 2001), pools that are storage-limited can be highly sensitive to climate variability. However, increasing heavy rainfall events may not necessarily result in increased pool persistence (particularly in pools closest to the location of rainfall) if subsurface storage up-gradient of the pool is already filling during the wet season. In this case, subsequent rainfall will increase streamflow downstream, but not result in increased subsurface storage in the reservoir supporting the pool. Moreover, recent work has shown that groundwater response times are sensitive to aridity, with longer response times associated with increased aridity (Cuthbert et al., 2019), so that there may be substantial time lags between climate variability and hydrologic response in pools supported by groundwater discharge.
We have distinguished between geological or topographic control on groundwater discharge, but this distinction may not always be critical from a management perspective. In any system connected to groundwater, perturbation of the dynamic equilibrium between groundwater recharge and discharge can impact surface water–groundwater interactions; the timing and extent of the change will depend on the magnitude and rate of alteration (Winter et al., 1998). The hydraulic head gradients (and groundwater discharge rates) supporting persistent river pools may be small (Δh on the order of centimetres), so that small decreases in groundwater level (either due to successive low-rainfall years or groundwater withdrawals) can potentially have a detrimental impact on the pool and cause the pool to dry out (particularly for topographically-controlled groundwater discharge to pools).
For pools supported by alluvial throughflow, the water balance is dominated by water outflow from contemporary fluvial deposits, but withdrawals from regional groundwater could impact the pool if these two subsurface reservoirs are hydraulically connected. The volume of groundwater storage in the source reservoir can indicate the resilience of pools to hydrological change (i.e. a longer groundwater system response time), but impacts will also depend on the distance from the recharge zone or groundwater withdrawals (which could include pumping or uptake by phreatophytes) (Cook et al., 2003). The time lag prior to a decrease in groundwater outflow to the pool, and shape of the response (i.e. a slow decline or sharp decrease), will also depend on the spatial distribution of the forcing (pool distance from recharge or groundwater withdrawals) (Cook et al., 2003; Manga, 1999). Thus, focussed groundwater withdrawals close to a pool will cause a larger and faster reduction in groundwater outflow than diffuse withdrawals across the aquifer, or withdrawals further away (Cook et al., 2003; Theis, 1940). For example, groundwater withdrawals from within 1 km of a pool will result in a rapid decrease in discharge (months to years), but the same volume of withdrawal distributed throughout the catchment will result in a more gradual decline in groundwater discharge to the pool (years to decades). Susceptibility can be further modified by geological barriers, which may not be obvious from the surface topography or regional geological maps (Bense et al., 2013), but can isolate pools from the regional groundwater system, and either (i) increase susceptibility to pumping within the connected aquifer, or (ii) reduce susceptibility if the pumping is on the other side of the barrier (Marshall et al., 2019).
Several tools in the hydrologist's toolbox are appropriate for gathering the data needed to distinguish between the hydraulic mechanisms that support pool persistence, as outlined in the previous section. For most of these, there are no examples in published literature that are specific to persistent pools along intermittent rivers. Therefore, this section provides general background and suggested considerations for the application of these methods to characterize the hydrology of persistent pools (Table 2). A selection of these tools may be deployed at a given site to characterize (a) the relationship of the pool to the groundwater system, and (b) the relative contributions of evaporation, transpiration, and groundwater fluxes (alluvial and regional) to the pool water balance.
Table 2Summary of pros and cons of available diagnostic tools for assessing the hydraulic mechanisms supporting persistent river pools.
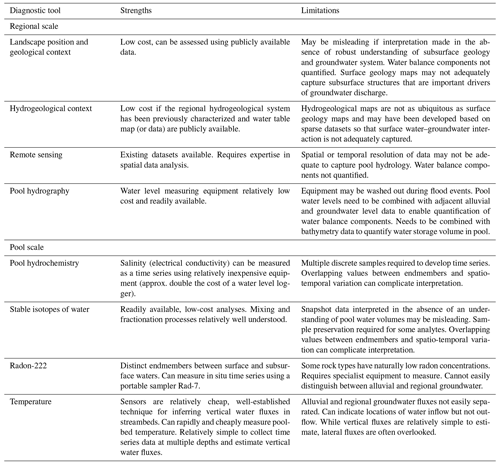
4.1 Regional-scale tools
4.1.1 Remote sensing and image analysis
Mapping the persistence of vegetation and water in the landscape based on remotely sensed data (i.e. Normalized Difference Vegetation Index (NDVI) or Normalized Difference Water Index (NDWI)) or aerial photos can be useful to identify river pools that persist in the absence of rainfall (Haas et al., 2009; Soti et al., 2009; Alaibakhsh et al., 2017). This can be valuable for identifying hydrologic assets that may require risk assessment and protection, but does not elucidate the hydraulic mechanism supporting the persistence of the pool. Interpretation of the key hydraulic mechanism(s) supporting a pool requires additional information on the landscape position and hydrogeological context of the pool. In combination, these regional-scale approaches are likely to be particularly useful in remote regions that are difficult to access and where pool-specific data collection is challenging.
4.1.2 Landscape position and geological context
Landscape position can provide some clues as to the mechanism controlling the persistence of a given pool. For example, a pool located high in the catchment on impermeable basement rock is likely to be a perched pool. Pools that reside within extensive alluvial deposits are likely to be supported at least in part by throughflow of alluvial groundwater. The presence or absence of alluvial deposits capable of hosting a significant volume of groundwater can often be determined by visual inspection, but geophysical tools or drilling are required to confirm the vertical extent of the alluvial aquifer. Similarly, a pool that is immediately prior to a topographic ridge that constrains the catchment is likely to be supported by geologically constrained groundwater discharge. Lateral catchment constriction can commonly be identified from publicly available aerial imagery, but identification of vertical catchment constriction will usually require geological data from drilling or regional-scale geophysical surveys. Aerial geophysics (e.g. AEM – airborne electromagnetics) in particular can aid in identifying subsurface lithologic geometries and low-permeability layers that can be important controls on groundwater outflow, but may not be obvious from aerial photographs or surface geology maps (Bourke et al., 2021). While the locations of geological can be evident from readily available maps of surface geology, the hydraulic properties of geological contacts are not always known a priori. Geological transitions can be zones of high permeability or barriers, or a combination of both (e.g. faults with high permeability in the vertical, and low permeability laterally) depending on the depositional and deformational history of the area (Bense et al., 2013).
4.1.3 Hydrogeological context
Regional groundwater mapping can provide insights into the mechanisms supporting persistent pools, particularly if the geology has also been well characterized (see case studies below for examples). Water table maps can articulate areas of groundwater recharge and discharge, and a step change in hydraulic head can be a key indicator for the presence of a hydraulic barrier (Fetter, 2001). If an interpreted water table surface suggests that the regional water table is tens of metres below ground in the vicinity of a pool, then the surface water is likely (but not definitely) perched. If a pool is situated in a region that has been identified as a regional groundwater discharge zone, then this groundwater discharge is likely to be supporting pool persistence. The presence of active deposition of geological precipitates can also be indicative of pool mode of occurrence, with carbonates associated with groundwater discharge and subsequent degassing of CO2 (Mather et al., 2019).
4.2 Pool-scale tools
4.2.1 Pool hydrography and water balance
If instrumentation can be installed in the pool, then it may be possible to characterize the pool water balance. Once a pool becomes isolated from the flowing river, a general pool water balance is given by , where V is the volume of water in the pool (L3), t is time (T), Qi is the water flux from the subsurface into the pool (L3 T−1), Qo is the water flux out of the pool into the subsurface (L3 T−1), E is the evapotranspiration rate (L T−1), and A is the surface area of the pool (L2). Here we neglect rainfall on the basis that a significant rainfall event is likely to initiate streamflow, but if this is not the case, then rainfall can be included as an additional term, PA, where P is the precipitation rate (L T−1). The water level in the pool, hp (L), can be routinely measured by installing pressure transducers, but conversion of water levels to pool water volume (and/or pool area) requires knowledge of pool bathymetry, and the relationship between hp and V will change during the dry season as the pool water level recedes (reducing the pool area, A) or if pool bathymetry is altered by scour and/or sediment deposition during flood events. Evapotranspiration rates can be taken from regional data or empirical equations, but actual losses can vary depending on solar shading, wind exposure, and transpiration (McMahon et al., 2016). For pools with visible surface inflow or outflow, these rates can potentially be measured using flow gauging (or dilution gauging), but relatively small flow rates and bifurcation of flow can make this challenging.
Modified versions of this general water balance can be defined for particular pools, depending on the hydraulic mechanism(s) supporting pool persistence (see Table 1). For perched pools, which are disconnected from the groundwater system, , so that the only component of the water balance is water loss through evaporation. Pools that are supported by alluvial throughflow are hydraulically connected to the water stored in the streambed alluvium. Water levels within this alluvium will be more dynamic than regional groundwater levels, so that influx and efflux rates that can change over time in response to rainfall events or seasonal drying (of the near subsurface). Where uptake of water by riparian vegetation from the groundwater store is substantial, this may need to be accounted for explicitly in a water balance model. For pools supported by groundwater discharge, influx will dominate over efflux (Qi > Qo). If the groundwater discharge is over an impermeable aquiclude (see Fig. 3b), there will commonly be a seepage zone up-gradient of the pool so that water influx is via surface inflow, but outflow to the subsurface can form a source of groundwater recharge to the adjacent (down-gradient) aquifer. If the groundwater discharge is controlled by topography, then the pool will be a site of regional groundwater discharge, so that local groundwater recharge (and Qo) should be negligible.
If a pool is connected to the groundwater system, Qi (or Qo) can be estimated from Darcy's Law; , where K is hydraulic conductivity, is the hydraulic gradient between the pool and the source aquifer, and Ai is the area over which the groundwater inflow occurs (which will usually be less than the total area of the base of the pool). The major limitations of this approach are that K of natural sediments varies by 10 orders of magnitude (Fetter, 2001) and that the area of groundwater inflow needs to be assumed or estimated using a secondary method. Hydraulic gradients between pools and streambed sediments can be measured using monitoring wells or temporary drive points, with Δh usually on the order of centimetres at most. Determination of the hydraulic gradient between regional aquifers requires that the water level in the pool has been surveyed to a common datum and there is a monitoring well near the pool to measure the groundwater level relative to that datum. In shallow, groundwater-dominated lakes, geophysical methods have also been used to determine local hydraulic gradients, and therefore the direction of the water flux(es) between groundwater and surface water (Ong et al., 2010; Befus et al., 2012). Blackburn et al. (2021) similarly applied shallow geophysical surveys, combined with mapping of hydraulic conductivities, to identify they key structures and processes controlling water fluxes between groundwater systems and the streams that host persistent pools (Blackburn et al., 2021).
4.2.2 Pool hydrochemistry and salinity (electrical conductivity)
Numerous studies of streams and lakes have employed hydrochemical and mass balance approaches to quantify water sources (Cook, 2013; Sharma and Kansal, 2013) and groundwater recharge (Scanlon et al., 2006). Some of these methods are also applicable in persistent pools, but may require modification or an iterative approach that allows for refinement of the methods as the mechanism supporting the pool is elucidated. In its simplest form, snapshot measurements of pool hydrochemistry (salinity, pH, major ions) can help distinguish pools that are connected to groundwater from those that are not (Williams and Siebert, 1963). Dissolved ions are relatively cheap and easy to measure, and have been used extensively to estimate recharge/discharge, groundwater flow, and ecohydrology in arid climates (Herczeg and Leaney, 2011). Electrical conductivity (EC) as an indicator of salinity can be measured at high temporal resolution using readily available loggers, which can be connected to telemetry systems if required. Time series of EC through flood-recession cycles can indicate relative rates of evaporation and throughflow (Siebers et al., 2016; Fellman et al., 2011), and allow identification of the hydraulic mechanism(s) supporting pool persistence. For example, if a pool is supported by regional groundwater discharge, the EC will re-equilibrate towards the groundwater EC value during the dry season (see case studies in Sect. 5); in a perched pool, the pool EC will not plateau, but continue to evapoconcentrate until the next flood event. However, in systems with large flood events, loggers can regularly become lost as the flood moves through, so EC loggers may need to be collected and downloaded prior to anticipated flood events, which is not always practical.
4.2.3 Stable isotopes of water
Stable isotopic values of pool water (δ18O and δ2H) can be interpreted similarly to electrical conductivity; groundwater seepage from a regional aquifer will have a relatively consistent isotopic value, while a pool isolated from the groundwater source will experience isotopic enrichment through evaporation (Hamilton et al., 2005), as demonstrated in case study 2 (Sect. 5.2.2). Pools receiving alluvial throughflow will have isotopic values that reflect the balance of inputs (from alluvial groundwater) and outputs (evapotranspiration and outflow to alluvial groundwater). However, the interpretation stable isotopic values can be limited by overlapping ranges of values across different water sources (Bourke et al., 2015) and spatiotemporal variability (see case studies). The isotopic values in the alluvial water itself can become enriched through evapotranspiration during the dry season resulting in variability over time, along the stream and throughout the catchment (Dogramaci et al., 2015), so that endmember values should be defined locally. In one case, strontium isotopes were found to be more useful than stable isotopes of water for identifying groundwater contributions to instream pools, because the strontium values in the groundwater endmember was far more constrained than salinity or stable isotope values (Bestland et al., 2017). Importantly, although these data are relatively easy to measure, their interpretation should ideally be supported by a robust understanding of the pool geometry, water flow paths, and the surrounding geology to ensure that the hydraulic mechanisms identified are physically plausible (see discussion).
4.2.4 Radon-222 and groundwater age indicators
Radon-222 is a commonly applied tracer in studies of surface water–groundwater interaction, and 222Rn mass balances have been effective for quantifying groundwater contributions to streams and lakes (Cook, 2013; Cook et al., 2008). Preliminary measurements of 222Rn in persistent pools indicate substantial spatial variability in 222Rn activity along the pools, reflecting the spatial distribution of groundwater influx and gas exchange. This spatial variability will limit quantification of groundwater discharge based on the 222Rn mass balance, but can allow for hotspots of groundwater discharge to be identified (see case studies).
Other groundwater age indicators (3H, 4He, 14C) have been measured along streams to identify groundwater sources (Gardener et al., 2011; Bourke et al., 2014b), but their applicability in pools is yet to be determined. Given that shallow, stagnant water is common, tracers such as 14C or 3H, which do not rapidly equilibrate with the atmosphere (Bourke et al., 2014b; Cook and Dogramaci, 2019), are likely to be better than gaseous isotopic tracers (e.g. 4He) that equilibrate rapidly (Gardener et al., 2011). If a mass balance approach is applied, then hydraulic measurements to constrain the pool water balance should be made in conjunction with hydrochemical sampling to ensure that the water balance is appropriately reflected in the mass balance.
4.2.5 Temperature as a tracer
Temperature measurements have been used extensively to identify and quantify water fluxes across streambeds and lake beds (e.g. Shanafield and Cook, 2014; Lautz, 2010). Diel amplitudes of subsurface temperatures have been used to identify the transition from flowing stream to dry channel (with isolated pools) in ephemeral systems (Rau et al., 2017). In persistent pools, temperatures at the water sediment interface can be used to map zones of groundwater inflow (Conant, 2004). In arid zones, groundwater temperatures will often be warmer than pool temperatures and this type of survey is best conducted at dawn when the temperature gradient between pool and groundwater is at a maximum and there are no confounding effects from direct solar radiation. This mapping can be conducted using point sensors or thermal cameras, but in natural water bodies this method has primarily found success at thermal springs where the temperature difference between surface waters and groundwater inflows is on the order of 10 ∘C (Briggs et al., 2016; Cardenas et al., 2011).
Vertical profiles of temperature can also be used to estimate vertical fluid fluxes, but the application of this approach in pools with coarse alluvial sediments (commonly throughflow pools) is likely to be limited by lateral flow within the subsurface when Kh > Kv (Rau et al., 2010; Lautz, 2010). Analytical solutions for temperature-based flux estimates also break down at low flux rates where the difference between convection and conduction is difficult to determine (Stallman, 1965). Recently developed instrumentation for measuring 3D flux fields (Banks et al., 2018) shows promise, but installation in course alluvial sediments like those commonly found in arid streambeds remains a challenge. Point-scale measurements also require upscaling, and these methods may not be applicable in fractured hard-rock pools.
In this section, we demonstrate the application of this framework to persistent river pools in north-west Australia (Fig. 5). Here we begin by providing an overview of the hydraulic mechanisms supporting persistent river pools in the Hamersley Basin based on regional-scale tools; landscape position and geological and hydrogeological context. We then present three pool-scale case studies to demonstrate how this contextual understanding can be supported by time series data from pools to further elucidate spatio-temporal variability in the key hydraulic mechanisms supporting pool persistence.
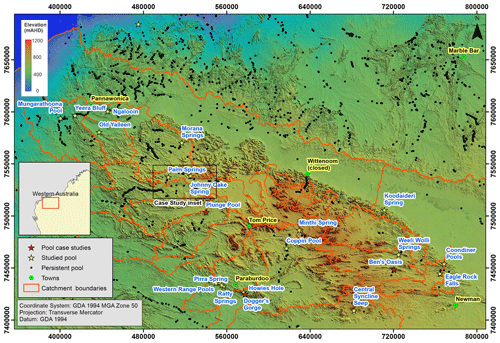
Figure 5Map of the prevalence of persistent pools on watercourses in the Hamersley Basin, and selected pools examined in detail. Persistent pools based on “waterholes” features from Geodata Topo 250 K Series 3 dataset; http://pid.geoscience.gov.au/dataset/ga/63999 (last access: 19 April 2021). Black rectangle indicates extent of Fig. 7.
The Hamersley Basin has an arid–tropical climate with a wet season from October to April and a dry season from May to September (Sturman and Tapper, 1996). Average annual rainfall is around 300 mm yr−1 with most rain falling between December and April (http://www.bom.gov.au, last access: 31 May 2021). Annual rainfall statistics can vary dramatically, depending on the influence of thunderstorms and cyclone activity. Thunderstorm activity is commonly highly localized, limiting the potential for spatial interpolation of data from individual monitoring sites. Annual evaporation is around 3000 mm yr−1 (http://www.bom.gov.au), or about 10 times annual rainfall, so that permanent surface water is rare. Ranges, spurs, and hills (consisting of bedrock and dikes described below) are separated by broad alluvial valleys with numerous deep gorges created by differential erosion. During large flood events, runoff creates sheet flow along the main channel, and the extensive floodplain can remain flooded for several weeks. In the absence of cyclonic rainfall, surface water is generally limited to a series of disconnected pools along the main channels. Bedrock consists of Archean basement rocks of the Weeli Wolli, Brockman, Wittenoom, and Marra Mamba formations (youngest to oldest) that are extensively folded and intruded by dolerite dikes (Table 3) with unconsolidated Tertiary and Quaternary sediments overlying them (Dogramaci et al., 2015). The valleys are filled with up to 100 m of consolidated and unconsolidated Tertiary detrital material consisting of clays, gravels, and chemical precipitates. The Quaternary alluvial sediments are deposited along the creek lines and incised channels (incised on the order of metres), and consist primarily of coarse, poorly sorted gravel, and cobbles (thickness of up to tens of metres, widths of up to hundreds of metres), resulting in relatively high hydraulic conductivity (see Table 3). The region is not considered to host regionally extensive, productive aquifers for water supply (Lau et al., 1987), but fresh groundwater is abundant throughout both within the Archean basement rocks, where permeability is increased via weathering, fracturing, or mineralization, and within the Tertiary and Quaternary sediments (Dogramaci et al., 2012). The geological basin has multiple surface water catchments with drainage flowing from the headwaters in the elevated areas towards the Fortescue, Robe, or Ashburton rivers.
Table 3Estimated horizontal hydraulic conductivities of geological units relevant to pool-scale case studies.
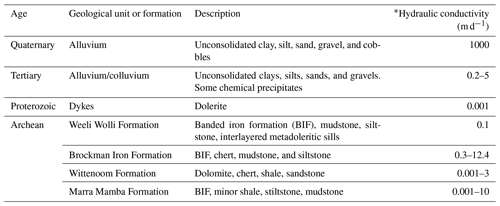
* Values from unpublished data and Dogramaci et al. (2015).
5.1 Regional-scale assessment of persistent pools within the Hamersley Basin
Regional-scale mapping of known pools has provided valuable insights about the distribution of pools and the likely hydraulic mechanisms supporting their persistence. Broad national-scale mapping of “waterholes” as part of the publicly available topographic dataset for this region identifies many pools along drainage lines but does not capture all of the known pools (see Fig. 5). National-scale groundwater-dependent ecosystem mapping is also available across Australia (http://www.bom.gov.au/water/groundwater/gde/service/simple-gde.php, last access: 13 February 2023). These data identify the groundwater dependence of some river reaches within the Hamersley Basin, but do not readily allow groundwater-dependent persistent pools to be differentiated from groundwater-dependent flowing streams. Image analysis and local knowledge has allowed for the identification of additional pools that were not mapped within the publicly available dataset.
Overlaying pool locations with topographic mapping allowed a number of pools located at points of lateral catchment constriction to be identified, suggesting that groundwater outflow (either alluvial or regional groundwater) supports pool persistence. The presence of a topographic constriction was confirmed using image analysis and direct observation (Fig. 6).
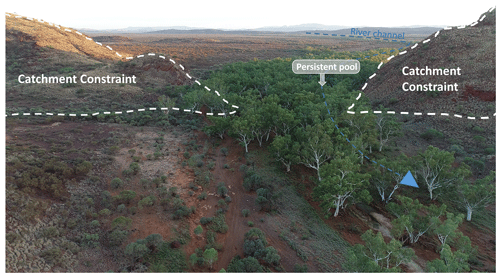
Figure 6Photo showing a river pool that persists where a surface catchment is constricted, resulting in a groundwater spring.
By overlaying the locations of pools with available maps of surface geology, we identified pools overlying elevated basement rock in the absence of an extensive alluvial channel, indicating the potential for these to be perched surface water (see the unnamed pools at the southern extent of Fig. 7 for example). For other pools, their location at the likely edge of a groundwater flow system where low-permeability basement intersected topography suggested regional groundwater outflow at geological contacts as an important hydraulic mechanism supporting persistence (e.g. Johnny Cake spring in Fig. 7). A number of pools were adjacent to mapped dikes; the persistence of these pools is potentially influenced by regional groundwater outflow to the surface facilitated by the dike acting as a hydraulic barrier within the subsurface. Other pools were located on mapped river-channel alluvium, indicating the likelihood that throughflow of alluvial groundwater at least partially supports pool persistence (e.g. pools along the contemporary flow path of Caves Creek in Fig. 7). The Hamersley Basin is a fractured-rock province that does not host aquifers that are used for large-scale water supply (Lau et al., 1987). As such, publicly available groundwater level data are sparse, and regional-scale mapping of water table or depth to groundwater contours that could further inform an assessment of the connectivity of pools to underlying groundwater is not available. NDVI mapping was undertaken (Fig. 7 inset); while this provides insights into persistence, it did not allow for the further elucidation of hydrological processes that may be driving this persistence.
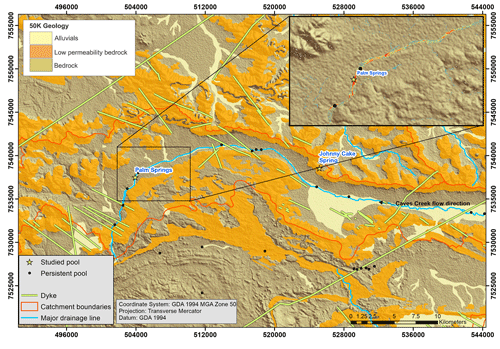
Figure 7Map showing locations of persistent pools along Caves Creek relative to bedrock, low-permeability bedrock, dikes, and alluvial river-channel sediments (geological mapping 50 K). Inset shows NDVI (on a scale of 0 in blue to 100 in red).
In situ observation of the landscape position of pools and the qualitative duration or pool persistence has also provided valuable insights into hydraulic mechanisms supporting pool persistence. For example, there are approximately 20 pools that reside within the ephemeral drainage lines of the Western Range that flow over hard rock for a few days in response to rainfall and do not have extensive alluvial deposits; a subset of these pools are deeply incised and shade persists all year round (Fig. 8a). The presence of an extensive alluvial deposit that would facilitate alluvial groundwater throughflow as a hydraulic mechanism was also able to be inferred based on surface geological mapping and direct visual observation (Fig. 8b). In other cases, alluvial deposits are present, and this alluvium is shaded within a gorge, so that alluvial throughflow is a major component of the water balance and evaporation is reduced relative to the alluvium outside the gorge, allowing surface water to persist (Fig. 8c).
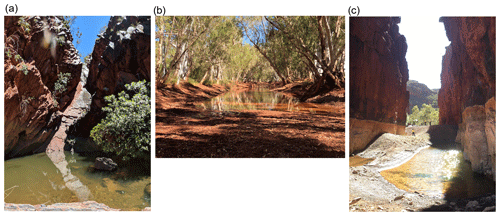
Figure 8Photos demonstrating presence or absence of alluvium indicating (a) no alluvium and perched surface water, (b) extensive alluvium and pool supported by throughflow of alluvial groundwater, and (c) alluvium within shaded gorge so that persistence of water sourced from outflow of alluvial groundwater is enhanced.
The exposure and outflow of regional groundwater as a mechanism supporting pool persistence was also able to be inferred from regional-scale datasets and direct observation in some cases. For example, some pools persist within deeply incised river gorges that do not contain extensive alluvial deposits and are therefore likely to be supported by topographically-controlled outflow of regional groundwater (Fig. 9a). In another case, groundwater was observed visibly seeping from an exposed rock face above a pool (Fig. 9b), inferring that the exposure of that geological unit at the surface and subsequent outflow of groundwater is important for supporting the persistence of that pool.
5.2 Pool-scale case studies
The following three case studies demonstrate the application of this framework to three different pools (or pool systems) within the Hamersley Basin. To the best of our knowledge, these pools have not been impacted by groundwater withdrawals or surface water diversions. With these case studies, we aim to demonstrate (a) the value of understanding the hydrogeological setting of each pool, and (b) spatio-temporal variability pool water balances and hydrochemistry. Each case study begins by describing our understanding of the landscape position and geological and hydrogeological context of the pool. We then introduce time series data; the first case study utilizes water levels and EC in the pool and groundwater, the second case study adds in time series of stable isotopes values of pool water and groundwater, the third case study brings in radon-222 and temperature mapping. By combining time series data with an understanding of landscape position and hydrogeological setting, we are able to infer hydraulic mechanisms supporting pool persistence. The implications of the identified hydraulic mechanisms for the susceptibility of the pools to groundwater withdrawals or changing climate are also discussed.
5.2.1 Case study 1: Plunge Pool
Plunge Pool (Fig. 10a) is located at the base of a steep topographic drop-off that exposes the Marra Mamba Formation. The Wittenoom Formation and underlying Marra Mamba Formation are hydraulically connected and form an unconfined regional aquifer where there has been sufficient weathering and fracturing to generate secondary porosity. This aquifer is 50–100 m thick and divided laterally by (sub)vertical dikes on the order of 1 km apart (but as close as 100 m) that act as hydraulic barriers within the groundwater system. The surface catchment has an area of approximately 26 km2 and is storage-limited. Regional groundwater in the adjacent aquifer has a hydraulic head of 547 m above sea level (a.s.l.) at a distance of 200 m from the pool, increasing to 557 m a.s.l. 600 m from the pool, indicating the presence of dike that acts as a geological barrier between these two monitoring wells. Seasonal variation in groundwater hydraulic heads is minimal (on the order of 0.2 m).
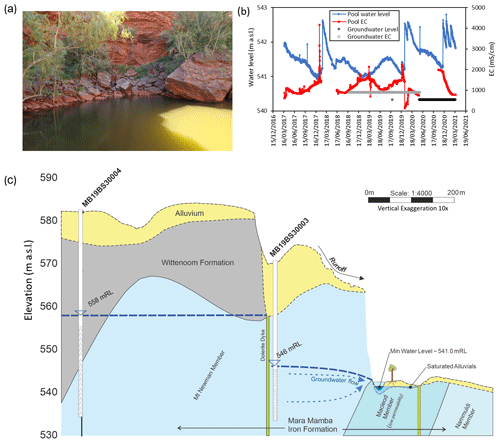
Figure 10(a) Photo of Plunge Pool, (b) pool water level and electrical conductivity (EC), and (c) hydrogeological setting of the pool.
The pool is perennial with seasonal water level fluctuation between 541 and 543 m a.s.l., driven by variation in streamflow, groundwater inflow, and evapotranspiration (Fig. 10b). The varying proportions of the pool water balance components are reflected in the temporal variation in the salinity of water in the pool. At the onset of the first wet season flood, the salinity in the pool spikes (up to 4171 mS cm−1), reflecting the flushing of surficial salts from across the catchment that accumulated during the dry season. Subsequent rainfall events then cause a rapid freshening of the pool (to as low as 124 mS cm−1 within 1 d). In the absence of rainfall, the salinity of the pool equilibrates to that of groundwater in the regional aquifer (900 mS cm−1). Given the consistency of groundwater levels, this inflow rate will be relatively constant, so that (in the absence of streamflow) the variability in the salinity of the pool is driven by seasonal variation in temperature and evapotranspiration (Bureau of Meteorology Station no. 007185, Paraburdoo Aero). These seasonal weather patterns drive evapoconcentration of solutes in the pool as water levels fall during the dry season and freshening of the pool as water levels rise when evapotranspiration decreases in winter (May–September). Measurement of the relationship between water levels and pool water volume will allow for these pool water balance components to be quantitatively resolved.
Based on these data, the dominant hydraulic mechanism supporting the persistence of this pool is attributed to groundwater inflow from the regional aquifer that is intersected by a topographic low (Sect. 2.3.2). Despite the source being a regional aquifer, the spatial extent of the groundwater reservoir supporting the pool is limited by the presence of geological dikes (Fig. 10c). The pool effectively acts as a “drain” on the underlying/adjacent compartment of the unconfined aquifer, with the inflow rate to the pool controlled by the hydraulic conductivity of the aquifer (variation in groundwater levels is negligible). The pool is also hydraulically connected to the alluvial aquifer, and water from the pool is likely to infiltrate into the alluvium on the down-gradient side, but this has not been measured directly (alluvium is absent up-gradient of the pool; therefore, alluvial throughflow is not a supporting mechanism). The susceptibility of this pool to groundwater withdrawals is controlled by the hydrogeological compartmentalization. The pool will be more susceptible to groundwater withdrawals from the aquifer between the nearest dike and the pool and less susceptible to groundwater withdrawals outside of this compartment. Given that evaporation is an important component of the water balance and contributes to the regulation of water levels, this pool is also susceptible to increases in evapotranspiration as temperatures increase under climate change (IPCC, 2022).
5.2.2 Case study 2: Howie's Hole
Howie's Hole is a pool within stream channel alluvium at the exit point of a short, narrow gorge (Fig. 11a). Immediately at the outlet of the gorge (approximately 30 m up-hydraulic-gradient of the pool), there is also a seep where groundwater outflows to surface for most of the year (seep dries for approximately 2–3 months at the end of the dry season). The seep is supported by the regional unconfined aquifer hosted within the Marra Mamba Formation and the surficial sediments above it (including the alluvial channel sediments), which are hydraulically connected. At the seep, the Brockman Formation has become adjacent to the Marra Mamba due to faulting, and this forms a relatively impermeable hydraulic barrier approximately 700 m wide (identified by the abrupt change in water table depth either side of the formation). The surface catchment upstream of Howie's Hole has an area of 33 km2. The gorge restricts the stream channel from 30 m width down to a channel width of 10 m, enhancing the water depth and flow rate, and resulting in scour and erosion of the Brockman Formation. This area of scour during high-flow events has subsequently been filled by deposition of unconsolidated alluvial sediments, which are now at the base of the pool (sediments speculated to be 5–10 m deep).
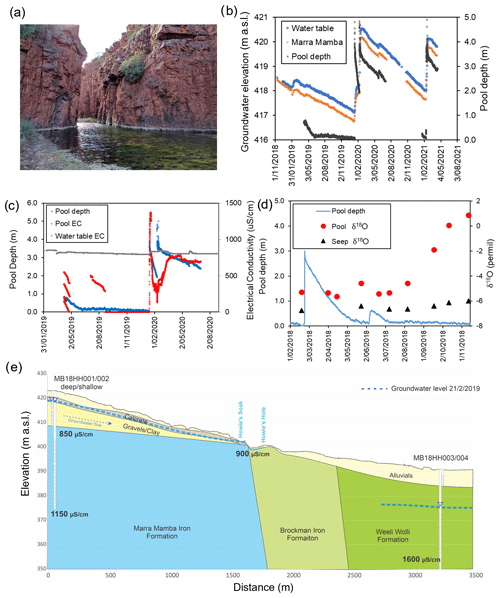
Figure 11(a) Photo of Howie's Hole, (b) groundwater elevations and pool depth, (c) pool water levels and electrical conductivity of pool and groundwater, (d) pool depth and δ18O showing stable isotopic composition at groundwater seep and evaporative enrichment down-gradient of seep during the dry season, and (e) conceptual diagram of pool occurrence.
The height of the regional water table is only known 1.5 km away from the seep, with seasonal fluctuations of 1–2 m (Fig. 11b). We assume that the water table declines towards the seep consistent with topographic elevation change (∼20 m drop over 1.5 km), and that the seep reflects the height of the water table at that location (elevation of the groundwater seep is 405 m a.s.l.). During the period of observation, the groundwater seep dried up when the measured water table elevation dropped below ∼ 418.4 m a.s.l. (water sample collected when the measured water table was at 418.5 m a.s.l. on 12 November 2018); the seep was dry when the measured water table was at 418.3 m a.s.l. on 7 December 2018. Pool water levels track groundwater elevations above 418 m a.s.l., but data from 2019 show the pool depth levelling off as the water table at the monitoring bore drops below 418 m a.s.l., suggesting the cessation of significant groundwater inputs. The pool water levels have not been surveyed to the Australian height datum, but pool water level is consistently below the elevation of the seep (approximately 398–400 m a.s.l.).
Similar to Plunge Pool, the pool salinity spikes with the seasonal onset of rainfall, before freshening once the accumulated salts have flushed through (Fig. 11c). In the absence of rainfall, pool salinity is similar to groundwater at the water table (Marra Mamba EC 1140 µS cm−1). Isotopic values were available for 2018 (which does not overlap with the EC data and water level data). During this dry season, isotope values of the seep and pool were relatively consistent until August when the pool isotopic values began to enrich, suggesting decreased inputs from groundwater as the water table receded (Fig. 11d).
Based on these data, we conclude that Howie's Hole reflects the water level in the alluvial aquifer within the stream channel (Fig. 11e). The location of the groundwater seep is determined by the geological contact between the permeable Marra Mamba Formation and impermeable Brockman Iron Formation in the subsurface, which coincides with the catchment constriction (gorge) that forms an outlet for surface and groundwater. As a result of the streamflow regime caused by this catchment constriction, the Brockman Iron Formation has been eroded and subsequently filled with unconsolidated stream channel sediments; water storage within these sediments now supports the persistence of this pool.
The water level and isotopic data indicate a threshold groundwater level for inflow of groundwater to the pool, such that the pool water balance is primarily dominated by groundwater recharged during the previous wet season. Below this threshold water level for groundwater inflow, the persistence of the pool relies on local water storage within the streambed alluvium (supporting pool depths of up to 0.2 m). The persistence of this pool is therefore susceptible to (1) wet season rainfall that is inadequate to recharge the unconfined aquifer to above the threshold water level, or (2) groundwater withdrawals that reduce seasonal peak groundwater levels to below the threshold level. In the absence of this groundwater inflow, the pool is supported by water stored locally within the streambed sediments (directly beneath the pool) and would be more susceptible to drying through evapotranspiration (less inflow but the same amount of water loss through evapotranspiration).
5.2.3 Case study 3: Ben's Oasis
Ben's Oasis is a sequence of three pools (pool 1, 2, and 3) that are hydraulically connected during peak water levels and subsequently disconnect during the dry season (Fig. 12a). The pools sit within a major drainage channel that consists of poorly sorted, fine to very coarse (gravel and boulders) unconsolidated alluvial sediments tens of metres wide and on the order of metres in thickness. The regional water table is within the fractured dolomite of the Wittenoom Formation, which overlies the Marra Mamba Formation. The pool is 2 km up-hydraulic-gradient of two parallel dikes, with a regional water table decline of approximately 20 m across these dikes, indicating that they act as a barrier within the groundwater system. Water levels in the upper pool have been monitored since 2016, and in 2019 a detailed study commenced using environmental tracers to assess the spatial variability of surface water–groundwater interaction along this pool sequence (Chapman, 2019).
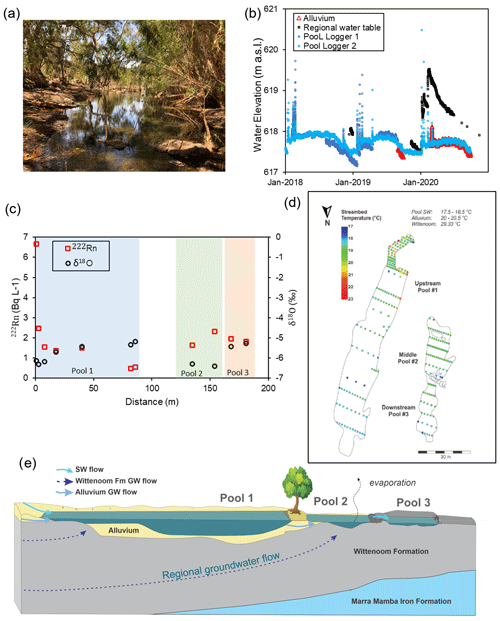
Figure 12(a) Photo of Ben's Oasis; (b) water levels in pool 1 (logger 1 elevation was surveyed, logger 2 elevation was approximated by matching data from logger 1), alluvium (DP1), and regional unconfined aquifer; (c) spatial variation in radon activities and δ18O along the pool sequence; (d) temperature mapping of pool sediments; and (e) conceptual diagram of mechanisms supporting pool persistence.
Measured pool water levels show consistent seasonal trends with water level spikes of 2–3 m in response to cyclonic rainfall events during summer, followed by approximately 5 months of relatively steady water levels and then recession over approximately 3 months (Fig. 12b). These trends are consistent with the water level variation in the adjacent alluvium, which exhibits a similar period of steady water levels then recession following the cessation of summer rains. In contrast, regional groundwater levels increase by about 2 m in response to summer rainfall and then immediately begin to recede. Thus, although snapshot water level measurements indicate that pool water levels are consistent with the regional water table, transient water level data (that include the water level in the alluvium) demonstrate that inflow of water from within the alluvial sediments within the drainage channel is the dominant driver of water level fluctuations in the upper pool (where the logger was installed). Spatial trends in the persistence of surface water and surface geology are also informative at this site. The regional Wittenoom aquifer is exposed at surface around pools 2 (some alluvium present) and 3 (no alluvium, just bedrock), but not at pool 1 (no bedrock, just alluvium). The upper, shallower section of pool 1 and pool 3 dried out as the dry season progressed, but the deeper parts of pools 1 and 2 persisted throughout the dry season (during 2019 and 2020). We interpret these spatial patterns of persistence as reflecting spatially variable evaporation rates (i.e. more or less shading by vegetation), transpiration rates, and groundwater inputs (Chapman, 2019).
The results of longitudinal hydrochemical surveys (222Rn and δ18O) along the pool sequence provide an independent line of evidence to validate this interpretation (Fig. 12c). Alluvial water had a 222Rn activity of 17.6 Bq L−1 and δ18O of −6.3 ‰. The regional Wittenoom aquifer had a lower 222Rn activity of 8.1 Bq L−1 and more depleted δ18O of −7.26 ‰. At the top of pool 1, 222Rn activity was 7 Bq L−1. Given that degassing of radon to the surface is rapid and the water level at the time of sampling was shallow, the source of water inflows must have a much higher 222Rn activity than 7 Bq L−1, and it is therefore most likely that inflows here are dominated by the higher Rn alluvial water. Isotopic δ18O values of around −6 ‰ are also consistent with inflow of alluvial water. 222Rn activities then decrease along the pool to around 0.5 Bq L−1 (indicating degassing and the absence of further groundwater inputs) as stable isotopic values enrich to just over −5 ‰ (reflecting evaporation and the absence of further groundwater inputs). Water at the upstream end of pool 2 had 222Rn of 2 Bq L−1 (greater than at the downstream end of pool 1) and δ18O of −6.3 ‰ (more depleted than at the bottom of pool 1). These data indicate further water inflows from the subsurface, along this pool, with a lesser proportion of alluvial water, and more regional groundwater, as well as throughflow from pool 1 (inferred from relative water levels in the pools). In pool 3, 222Rn remains around 2 Bq L−1, indicating further groundwater inputs, but the stable isotopic values are more enriched (possibly due to the shallow water depth allowing for enhanced evaporation).
Streambed temperatures within the pools were also mapped (temperatures measured every 0.2–1 m along transects 1–10 m apart) in early September, when regional groundwater was 29 ∘C and alluvial water was 20 ∘C (Fig. 12d). Measured temperatures were recorded at dawn to reduce the effect of direct solar radiation and pool depth variability (max pool depth was 0.5 m). Streambed temperatures in the pools ranged from 17–23 ∘C, with the warmest water (> 20 ∘C) at the top of pool 1, and temperatures between 19–20 ∘C in middle of pool 2 and at the top of pool 3. These results are broadly consistent with the other results, but the approach is likely to be more conclusive in the presence of larger temperature gradients. The application of vertical temperature profiles to infer water fluxes at this site was also limited by the substantial lateral component of the subsurface flow field (i.e. violating the assumption of 1D flow) and flood events that removed or damaged monitoring infrastructure.
Based on these data, we conclude that the persistence of Ben's Oasis throughout the dry season is supported by regional groundwater inflows from the unconfined aquifer where it is exposed at surface (see Sect. 2.3), but the water balance of pool 1 is dominated by exchange with the alluvial water (see Sect. 2.2). This importance of the alluvial water storage in supporting the largest of these pools is only evident based on time series water level data from the alluvium. Given only snapshot water level measurements from the regional aquifer and one location in the pools, the similarity in water level elevations would lead to the conclusion that regional groundwater discharge was the dominant supporting mechanism. The substantial spatial variability captured in the longitudinal hydrochemical survey also highlights the risks of making conclusions about surface water–groundwater interactions from snapshot hydrochemistry measurements in just one location within a given pool or pool sequence. Subsequent numerical modelling of the groundwater system indicates that the presence of the regional-scale dikes east of the pool operates as a hydraulic barrier within the groundwater system, supporting the regional water table west of the dikes, promoting regional groundwater outflow to the surface at the pool (Jen Gleeson, personal communication, 2022).
These data allow us to infer that there are two hydraulic mechanisms supporting the water balance and persistence of these pools; alluvial throughflow and regional groundwater discharge (Fig. 12e). The persistence of these pools through the dry season is dependent on influx of water from the regional unconfined aquifer. They will therefore be susceptible to groundwater withdrawals from the regional aquifer if they reduce the hydraulic head to below the level of the ground surface at the pools. The water balance of these pools is also controlled by the interaction with water stored in the alluvium (alluvial throughflow). Therefore, the pools are also susceptible to reductions in rainfall or increases in temperature (and evapotranspiration) that reduce the volume of water storage (and therefore water levels) within the streambed alluvium. A reduction in the area of the surface catchment, which can result from mining operations, could also similarly alter the water balance of these pools.
It has now been 100 years since groundwater springs were documented in published literature (Bryan, 1919; Meinzer, 1927, 1923). In that time, the literature on springs, surface water–groundwater interactions, and non-perennial rivers have all expanded considerably. The goal of the present work has been to synthesize concepts from all of those fields to aid in the identification of hydraulic mechanisms that support instream pools. Thus, in Sect. 2, we identified four primary pool types, discussing hydraulic mechanisms for each conceptually and identifying relevant background literature to support each. In Sect. 4, we then provide a toolbox for use on individual pools and at the regional scale, and show in Sect. 5 how this toolbox can be used through a series of case studies. This identification of the hydraulic mechanisms is essential for effective management of risks to pool ecosystems associated with groundwater withdrawals, changes to the hydraulic properties of the catchment (e.g. land use change), or climate change, as discussed in Sect. 3.
Across the three case studies, the persistence of all pools was related to geological contacts that resulted in regional groundwater outflow. Plunge Pool and Howie's Hole are both located where a low-permeability geological unit results in groundwater outflow to the surface; the water cannot easily continue to move in the subsurface, so it emerges at the point of contact. At Ben's Oasis, there is regional groundwater outflow where saturated fractured rock is exposed at the surface and the hydraulic head is above the land surface. In all of these cases, there were additional hydraulic mechanisms supporting pool persistence. At Plunge Pool, the geological contact coincides with a topographic low; at Howie's Hole, there is a catchment constriction; at Ben's Oasis, alluvial throughflow is a key determinant of pool water levels. Thus, the water balance of persistent pools can respond to a combination of hydraulic mechanisms, and the dominant mechanisms can vary spatially and temporally within pools. In all three cases, the pool(s) contain a mixture of water from streambed sediments (alluvial throughflow) and regional groundwater during certain hydroperiods, but the pool likely would not persist through the dry season in that location without groundwater discharge from the regional aquifer. Thus, the maintenance of the stream ecosystem in its current state would require preservation of instream water storage and regional groundwater inflows. Such pools combining alluvial throughflow pools with some regional groundwater input are likely common, but it can be difficult to definitively identify and/or quantify this regional component of the water balance.
Given the potential for this complexity, we advocate for the use of multiple lines of evidence in determining hydraulic mechanisms, in line with the accepted paradigm in surface water–groundwater interactions literature (Kalbus et al., 2006). Regional-scale tools provide a valuable method to make a first estimate of hydraulic mechanisms; however, highly instrumented sites with robust geological mapping, monitoring wells, and temporal hydrologic data are required to elucidate spatio-temporal variability in the pool water balance. Likewise, snapshot data from multiple pools at one point in time can help distinguish perched pools vs. groundwater discharge pools (i.e. pool water hydrochemically similar or different to rainfall or groundwater), but in some cases, water types (or endmembers) are difficult to distinguish based on easily measured parameters like electrical conductivity or stable isotopes of water (Bourke et al., 2015).
However, we also acknowledge that direct measurement of water balances in arid and semi-arid regions can be logistically difficult (Villeneuve et al., 2015). Rainfall (and therefore runoff) in arid and semi-arid environments is commonly patchy and water fluxes can be either too large to measure (streamflow during a cyclone) or too small to measure directly (dry season groundwater seepage fluxes) (Shannon et al., 2002; Shanafield and Cook, 2014). There are also potential logistical constraints that can apply when installing any infrastructure for sampling and monitoring instream pools. Persistent pools in arid landscapes are commonly sites of environmental and cultural significance (Finn and Jackson, 2011; Yu, 2000), so that appropriate approvals and permissions typically must be obtained prior to the installation of monitoring infrastructure. This may restrict the types of data that can be collected. Moreover, some sites may be sacred sites, limiting who is able to access them. Surface water features in general are a draw for travellers and roaming livestock, so that any infrastructure must be secure from theft or damage. Flood events and sudden, flashy streamflows are also potential threats to infrastructure, with substantial sediment and vegetation (branches, trees) transported across the floodplain to heights of 2–3 m that can (and have) destroyed sampling equipment. Infrastructure damage by unseasonal or early rainfalls in particular can impact our ability to capture regional groundwater contributions, since this is typically a relatively small (but important) component of the water balance of pools and is most readily captured at the end of the dry season.
With limited resources and access to sites, trade offs must therefore be made between detailed characterization of one pool vs. a minimal dataset at many pools. In our experience, utilizing detailed data from fewer pools is more likely to provide a robust characterization of pool hydrology at a scale required for management than snapshot data from many pools across a region, which can be open to misinterpretation. For example, while there is no isotopic fractionation associated with the outflow of water from a pool, there is mass removal of water (and solutes) from the pool. As such, this water loss term should be accounted for when interpreting measured isotopic values of a pool. This is because the change in isotopic value (or solute concentration, C) over time () of any water body is a function of the water balance and the mass balance (Cook, 2013; Bourke et al., 2014b). Errors in the water balance will therefore propagate through to errors in the interpretation of measured concentration data.
This point can be demonstrated using a simple synthetic model of a pool with a surface area of 200 m2 and a depth of 2 m. The change in pool volume and δ18O over 112 d was simulated using the method and parameter values of Bourke et al. (2021) for four different water balance configurations (Fig. 13). In the first scenario, evapotranspiration is the only loss (or gain) term in the water balance. Evaporation is set at 0.007 m d−1 and results in isotopic fractionation; transpiration is set at 0.003 m d−1 and there is no associated fractionation (total loss rate 0.01 m d−1). In the second scenario, groundwater inflows of 2.88 m3 d−1 are added with the δ18O of groundwater set at −7 ‰. In the third scenario, groundwater inflow and outflow are implemented (as well as evapotranspiration) with a net groundwater inflow of 2.88 m3 d−1 (groundwater inflow of 11.52 m3 d−1 and outflow of 8.64 m3 d−1. The simulated pool volumes are identical in scenarios 2 and 3, but the isotopic values are different because the water balance equations are different, and therefore the equation for dC dt is different in scenario 2, as compared to scenario 3. Thus, if the pool water outflow term was neglected when interpreting measured stable isotope values from the pool, the results would be inaccurate. And although the difference in isotopic enrichment may be small under different water balance scenarios, the cumulative impact could still be important hydro-ecologically (the associated error is 8–30 % of initial pool water balance in scenarios shown below). The fourth scenario has the same evapotranspiration and groundwater inflow as scenario 2, but the pool geometry is changed so that the pool area is halved and the depth is doubled (A= 100 m2, d= 4 m). This scenario demonstrates that the isotopic evolution of this hypothetical pool is very sensitive to the surface area to volume ratio of the pool; and yet these geometric properties can be difficult to characterize and are rarely reported in published literature (they are also lacking in the case studies presented herein).
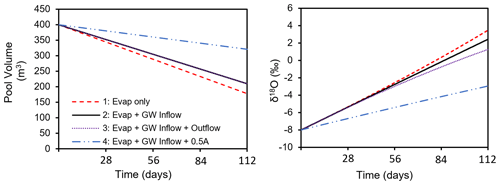
Figure 13Evolution of pool volume and values of stable isotopes of water in pools with varying water balance components over approximately 4 months of dry season (evap: evapotranspiration, GW: groundwater, A: pool area). Model modified after Bourke et al. (2021) to simulate a pool instead of a flowing stream.
Such potential pitfalls can be found in all single methods. Thus, we suggest an initial regional-scale assessment of landscape position and hydrogeological context that allows for pools to be grouped into likely hydraulic mechanisms; a representative subset of these can be instrumented and sampled to provide time series of water levels (groundwater and surface water) and hydrochemistry to understand the pool water balance.
This was our approach in the Hamersley Basin, and the above points can be seen in our results. We were able to identify key hydraulic mechanisms supporting pool persistence at a number of pools at regional and local scale. However, the spatio-temporally variable components of the water balance remain difficult to constrain. Although there is a lot of data in the region overall, given the remote and inaccessible nature of these pools, none of them have a complete dataset of the kind advocated for here. It should be noted that as with every field study, these case studies do not represent perfect examples of the hypothetical cases, but are instead limited by typical considerations found in the real world and are subject to ongoing research efforts. In particular, Ben's Oasis provides an example of a pool that is particularly difficult to characterize and cannot simply be linked to one hydraulic mechanism. Efforts to characterize the bathymetry of Ben's Oasis have been fraught with challenges, and the relationship between water level and pool volume remains uncertain, limiting our efforts to confidently determine the water balance.
In this work, we have striven to provide a useful framework, based on a conceptual, first-principles understanding, supported by both useful tools and case studies. However, this has also resulted in limitations. Each of these topics could be presented as a full study. The list of field and regional-scale methods is not exhaustive, but instead presents the most commonly used and accessible tools. Various other tools, such as geophysical surveys and varied geochemical tracers, could easily be employed to garner additional data useful in further understanding instream pool hydrology. Moreover, the review of supporting literature, in particular from the field of groundwater–surface water interactions, has been necessarily concise and more could be said. However, we feel that there is utility in presenting the basic background in conjunction with field tools and considerations, allowing each reader can take the parts that are most relevant to their own needs and seek out further background from the cited literature as needed. We hope this work serves as a common platform for a deeper understanding of instream pools globally, as non-perennial streams are increasingly recognized for both their importance and their vulnerability in our changing world.
The study of persistent river pools is a developing science and much remains to be done. Policymakers increasingly require accurate information on the mode of occurrence of surface water pools to put forward management plans to mitigate and/or minimize the adverse impacts of human activities (Leibowitz et al., 2008). This framework is subject to refinement as sufficient data become available to fully characterize pool water balances and mode of occurrence. Extension of this framework to facilitate the incorporation of biological and sedimentological processes is also desirable. Persistent river pools exist in all climates across the globe, and consistent data on geomorphology, hydrology, and ecology should be collected at multiple features so that generalized patterns and processes can be elucidated. The nutrient and carbon transport between pools during flows and the effects of anthropogenic disruption to groundwater inputs or surface water flushes into these pools is also not well known. These disruptions can be detrimental to water quality if the anthropogenic inputs are contaminated (Jackson and Pringle, 2010), but may also support seasonal connectivity that benefits the ecosystem by distributing nutrients and organic matter between pools (Jaeger et al., 2014). Effects of climate change (e.g. lower groundwater levels, thermal loading, and altered storm cycles) also combine with geomorphological and biological factors to impact ecosystem function, but these mechanisms are not yet well understood.
Persistent pools are an important feature along non-perennial rivers and these types of systems are under increasing pressure from altered hydrology associated with shifting climates and anthropogenic activities (Steward et al., 2012). Three dominant hydraulic mechanisms that support the persistence of river pools were identified from literature on groundwater springs and groundwater–surface water interaction; perched surface water, throughflow of alluvial water, and regional groundwater discharge. Regional groundwater discharge can be further characterized into two types of control on groundwater outflow; geological barrier vs. topography. While the existing literature hints at the hydrologic and geologic constraints imperative to pool persistence, the framework presented here provides cohesive synthesis of hydraulic mechanisms supporting persistence, as required to sufficiently understand and protect persistent river pools globally. Susceptibility to hydrological change depends on the mechanism(s) of pool persistence and the spatial distribution of stressors relative to the pool. Further research is required to resolve the impacts hydroclimatic stressors at the scale of individual pools.
A suite of diagnostic tools are available for understanding the hydrologic mechanisms that support the persistence of a given river pool. A regional-scale assessment can be made based on an understanding of the pool's landscape position and hydrogeological context, which may be supported by remote sensing or image analysis. Time series data of water levels and hydrochemistry are required to resolve the spatiotemporal variability in pool water balances, as demonstrated in the three pool-scale case studies presented. The suitability of each of these tools to any given pool or study will depend on the data and resources available, and the requirement for a coarse or highly detailed resolution of the mechanisms supporting pool persistence.
The data used in Sect. 5 of this paper are the property of Rio Tinto. Access to these data may be requested by contacting Paul Hedley (paul.hedley2@riotinto.com).
SAB and MS prepared the text of the paper with input from all co-authors. PH, SC, SD, and SAB collected and analysed the data presented in Sect. 5. PH and SAB prepared the figures.
The contact author has declared that none of the authors has any competing interests.
Publisher’s note: Copernicus Publications remains neutral with regard to jurisdictional claims in published maps and institutional affiliations.
We thank the editors and reviewers of this paper and the preceding submission (https://doi.org/10.5194/hess-2020-133) for providing constructive comments that allowed us to substantially improve the paper prior to publication. We particularly want to thank the two student reviewers who provided exceptionally detailed and useful critique – may you receive similarly useful reviews of your own work.
Author Shanafield's contribution was supported by funding from the Australian Research Council (grant no. DE150100302).
This paper was edited by Patricia Saco and reviewed by Francesc Gallart and one anonymous referee.
Alaibakhsh, M., Emelyanova, I., Barron, O., Khiadani, M., and Warren, G.: Large-scale regional delineation of riparian vegetation in the arid and semi-arid Pilbara region, WA, Hydrol. Process., 31, 4269–4281, 2017.
Alfaro, C. and Wallace, M.: Origin and classification of springs and historical review with current applications, Environ. Geol., 24, 112–124, https://doi.org/10.1007/bf00767884, 1994.
Arthington, A. H., Balcombe, S. R., Wilson, G. A., Thoms, M. C., and Marshall, J.: Spatial and temporal variation in fish-assemblage structure in isolated waterholes during the 2001 dry season of an arid-zone floodplain river, Cooper Creek, Australia, Mar. Freshwater Res., 56, 25–35, https://doi.org/10.1071/MF04111, 2005.
Ashley, G. M., Goman, M., Hover, V. C., Owen, R. B., Renaut, R. W., and Muasya, A. M.: Artesian blister wetlands, a perennial water resource in the semi-arid rift valley of East Africa, Wetlands, 22, 686–695, 2002.
Banks, E. W., Shanafield, M. A., Noorduijn, S., McCallum, J., Lewandowski, J., and Batelaan, O.: Active heat pulse sensing of 3-D-flow fields in streambeds, Hydrol. Earth Syst. Sci., 22, 1917–1929, https://doi.org/10.5194/hess-22-1917-2018, 2018.
Befus, K. M., Cardenas, M. B., Ong, J. B., and Zlotnik, V. A.: Classification and delineation of groundwater–lake interactions in the Nebraska Sand Hills (USA) using electrical resistivity patterns, Hydrogeol. J., 20, 1483–1495, https://doi.org/10.1007/s10040-012-0891-x, 2012.
Bense, V. F., Gleeson, T., Loveless, S. E., Bour, O., and Scibek, J.: Fault zone hydrogeology, Earth-Sci. Rev., 127, 171–192, https://doi.org/10.1016/j.earscirev.2013.09.008, 2013.
Bestland, E., George, A., Green, G., Olifent, V., Mackay, D., and Whalen, M.: Groundwater dependent pools in seasonal and permanent streams in the Clare Valley of South Australia, J. Hydrol.-Regional Studies, 9, 216–235, 2017.
Bishop-Taylor, R., Tulbure, M. G., and Broich, M.: Surface-water dynamics and land use influence landscape connectivity across a major dryland region, Ecol. Appl., 27, 1124–1137, https://doi.org/10.1002/eap.1507, 2017.
Blackburn, J., Comte, J., Foster, G., and Gibbins, C.: Hydrogeological controls on the flow regime of an ephemeral temperate stream flowing across an alluvial fan, J. Hydrol., 595, 125994, https://doi.org/10.1016/j.jhydrol.2021.125994, 2021.
Bogan, M. T. and Lytle, D. A. Severe drought drives novel community trajectories in desert stream pools, Freshwater Biol., 56, 2070–2081, https://doi.org/10.1111/j.1365-2427.2011.02638.x, 2011.
Bonada, N., Cañedo-Argüelles, M., Gallart, F., von Schiller, D., Fortuño, P., Latron, J., Llorens, P., Murria, C., Soria, M., Vinyoles, D., and Cid, N.: Conservation and management of isolated pools in temporary rivers, Water, 12, 2870, https://doi.org/10.3390/w12102870, 2020.
Boulton, A. J.: Parallels and contrasts in the effects of drought on stream macroinvertebrate assemblages, Freshwater Biol., 48, 1173–1185, https://doi.org/10.1046/j.1365-2427.2003.01084.x, 2003.
Bourke S. A., Cook, P. G., Shanafield, M., Dogramaci, S., and Clark, J. F.: Characterisation of hyporheic exchange in a losing stream using radon-222, J. Hydrol., 519, 94–105, https://doi.org/10.1016/j.jhydrol.2014.06.057, 2014a.
Bourke, S. A., Harrington, G. A., Cook, P. G., Post, V. E., and Dogramaci, S.: Carbon-14 in streams as a tracer of discharging groundwater, J. Hydrol., 519, 117–130, https://doi.org/10.1016/j.jhydrol.2014.06.056, 2014b.
Bourke, S. A., Cook, P. G., Dogramaci, S., and Kipfer, R.: Partitioning sources of recharge in environments with groundwater recirculation using carbon-14 and CFC-12, J. Hydrol., 525, 418–428, 2015.
Bourke, S. A., Degens, B., Searle, J., de Castro Tayer, T., and Rother, J.: Geological permeability controls streamflow generation in a remote, ungauged, semi-arid drainage system, J. Hydrol.-Regional Studies, 38, 100956, https://doi.org/10.1016/j.ejrh.2021.100956, 2021.
Brooks, R. T. and Hayashi, M.: Depth-area-volume and hydroperiod relationships of ephemeral (vernal) forest pools in southern New England, Wetlands, 22, 247–255, https://doi.org/10.1672/0277-5212(2002)022[0247:DAVAHR]2.0.CO;2, 2002.
Briggs, M. A., Hare, D. K., Boutt, D. F., Davenport, G., and Lane, J. W.: Thermal infrared video details multiscale groundwater discharge to surface water through macropores and peat pipes, Hydrol. Process., 30, 2510–2511, https://doi.org/10.1002/hyp.10722, 2016.
Brunner, P., Cook, P., and Simmons, C.: Hydrogeologic controls on disconnection between surface water and groundwater, Water Resour. Res., 45, W01422, https://doi.org/10.1029/2008WR006953, 2009.
Bryan, K.: Classification of springs, J. Geol., 27, 522–561, 1919.
Bunn, S. E., Thoms, M. C., Hamilton, S. K., and Capon, S. J.: Flow variability in dryland rivers: boom, bust and the bits in between, River Res. Appl., 22, 179–186, https://doi.org/10.1002/rra.904, 2006.
Caldwell, T. G., Wolaver, B. D., Bongiovanni, T., Pierre, J. P., Robertson, S., Abolt, C., and Scanlon, B. R.: Spring discharge and thermal regime of a groundwater dependent ecosystem in an arid karst environment, J. Hydrol., 587, 124947, https://doi.org/10.1016/j.jhydrol.2020.124947, 2020.
Cardenas, M. B. and Wilson, J. L.: Exchange across a sediment–water interface with ambient groundwater discharge, J. Hydrol., 346, 69–80, https://doi.org/10.1016/j.jhydrol.2007.08.019, 2007.
Cardenas, M. B., Neale, C. M. U., Jaworowski, C., and Heasler, H.: High-resolution mapping of river-hydrothermal water mixing: Yellowstone National Park, Int. J. Remote Sens., 32, 2765–2777, https://doi.org/10.1080/01431161003743215, 2011.
Chapman, S.: Groundwater discharge to persistent in-stream pools in dryland regions, Master's Thesis, School of Earth Sciences, University of Western Australia, 2019.
Conant, B.: Delineating and quantifying ground water discharge zones using streambed temperatures, Ground Water, 42, 243–257, 2004.
Cook, P. G.: Estimating groundwater discharge to rivers from river chemistry surveys, Hydrol. Process., 27, 3694–3707, https://doi.org/10.1002/hyp.9493, 2013.
Cook, P. G. and Dogramaci, S.: Estimating Recharge From Recirculated Groundwater With Dissolved Gases: An End-Member Mixing Analysis, Water Resour. Res., 55, 5468–5486, https://doi.org/10.1029/2019wr025012, 2019.
Cook, P. G., Jolly, I. D., Walker, G. R., and Robinson, N. I.: From drainage to recharge to discharge: Some timelags in subsurface hydrology, Dev. Water Sci., 50, 319–326, 2003.
Cook, P. G., Wood, C., White, T., Simmons, C. T., Fass, T., and Brunner, P.: Groundwater inflow to a shallow, poorly-mixed wetland estimated from a mass balance of radon, J. Hydrol., 354, 213–226, https://doi.org/10.1016/j.jhydrol.2008.03.016, 2008.
Costigan, K. H., Daniels, M. D., and Dodds, W. K.: Fundamental spatial and temporal disconnections in the hydrology of an intermittent prairie headwater network, J. Hydrol., 522, 305–316, https://doi.org/10.1016/j.jhydrol.2014.12.031, 2015.
Costigan, K. H., Jaeger, K. L., Goss, C. W., Fritz, K. M., and Goebel, P. C.: Understanding controls on flow permanence in intermittent rivers to aid ecological research: integrating meteorology, geology and land cover, Ecohydrology, 9, 1141–1153, https://doi.org/10.1002/eco.1712, 2016.
Cranswick, R. H. and Cook, P. G.: Scales and magnitude of hyporheic, river-aquifer and bank storage exchange fluxes, Hydrol. Process., 29, 3084–3097, 2015.
Cushing, C. E. and Wolf, E. G.: Primary production in Rattlesnake Springs, a cold desert spring-stream, Hydrobiologia, 114, 229–236, https://doi.org/10.1007/bf00031874, 1984.
Cuthbert, M. O., Gleeson, T., Reynolds, S. C., Bennett, M. R., Newton, A. C., McCormack, C. J., and Ashley, G. M.: Modelling the role of groundwater hydro-refugia in East African hominin evolution and dispersal, Nat. Commun., 8, 1–11, 2017.
Cuthbert, M. O., Gleeson, T., Moosdorf, N., Befus, K. M., Schneider, A., Hartmann, J., and Lehner, B.: Global patters and dynamics of climate-groundwater interactions, Nat. Clim. Change, 9, 137–141, 2019.
Davis, L., Thoms, M. C., Fellows, C., and Bunn, S.: Physical and ecological associations in dryland refugia: waterholes of the Cooper Creek, Australia, International Association of Hydrological Sciences, Publication, 276, 77–84, 2002.
Deemy, J. B. and Rasmussen, T. C.: Hydrology and water quality of isolated wetlands: Stormflow changes along two episodic flowpaths, J. Hydrol.-Regional Studies, 14, 23–36, https://doi.org/10.1016/j.ejrh.2017.10.001, 2017.
DelVecchia, A. G., Shanafield, M., Zimmer, M. A., Busch, M. H., Krabbenhoft, C. A., Stubbington, R., Kaiser, K. E., Burrows, R. M., Hosen, J., Datry, T., and Kampf, S. K.: Reconceptualizing the hyporheic zone for nonperennial rivers and streams, Freshwater Sci., 41, 167–182, https://doi.org/10.1086/720071, 2022.
Doble, R., Brunner, P., McCallum, J., and Cook, P. G.: An analysis of river bank slope and unsaturated flow effects on bank storage, Groundwater, 50, 77–86, https://doi.org/10.1111/j.1745-6584.2011.00821.x, 2012.
Dogramaci, S., Skrzypek, G., Dodson, W., and Grierson, P. F.: Stable isotope and hydrochemical evolution of groundwater in the semi-arid Hamersley Basin of subtropical northwest Australia, J. Hydrol., 475, 281–293, 2012.
Dogramaci, S., Firmani, G., Hedley, P., Skrzypek, G., and Grierson, P. F.: Evaluating recharge to an ephemeral dryland stream using a hydraulic model and water, chloride and isotope mass balance, J. Hydrol., 521, 520–532, 2015.
Fellman, J. B., Dogramaci, S., Skrzypek, G., Dodson, W., and Grierson, P. F.: Hydrologic control of dissolved organic matter biogeochemistry in pools of a subtropical dryland river, Water Resour. Res., 47, W06501, https://doi.org/10.1029/2010wr010275, 2011.
Fetter, C. W.: Applied hydrogeology (Fourth Edition), Prentice Hall, Upper Saddle River, USA, ISBN 10 1-292-02290-6, ISBN 13 978-1-292-02290-1, 2001.
Finn, M. and Jackson, S.: Protecting Indigenous Values in Water Management: A Challenge to Conventional Environmental Flow Assessments, Ecosystems, 14, 1232–1248, https://doi.org/10.1007/s10021-011-9476-0, 2011.
Gardener, W. P., Harrington, G. A., Solomon, D. K., and Cook, P. G.: Using terrigenic 4He to identify and quantify regional groundwater discharge to streams, Water Resour. Res., 47, W06523, https://doi.org/10.1029/2010WR010276, 2011.
Gibson, J. J.: Short-term evaporation and water budget comparisons in shallow Arctic lakes using non-steady isotope mass balance, J. Hydrol., 264, 242–261, 2002.
Godsey, S. E. and Kirchner, J. W.: Dynamic, discontinuous stream networks: hydrologically driven variations in active drainage density, flowing channels and stream order, Hydrol. Process., 28, 5791–5803, https://doi.org/10.1002/hyp.10310, 2014.
Goodrich, D. C., Kepner, W. G., Levick, L. R., and Wigington Jr., P. J.: Southwestern Intermittent and Ephemeral Stream Connectivity, JAWRA J. Am. Water Resour. As., 54, 400–422, https://doi.org/10.1111/1752-1688.12636, 2018.
Gutiérrez-Jurado, K. Y., Partington, D., Batelaan, O., Cook, P., and Shanafield, M.: What Triggers Streamflow for Intermittent Rivers and Ephemeral Streams in Low-Gradient Catchments in Mediterranean Climates, Water Resour. Res., 55, 9926–9946, https://doi.org/10.1029/2019WR025041, 2019.
Haas, E. M., Bartholomé, E., and Combal, B.: Time series analysis of optical remote sensing data for the mapping of temporary surface water bodies in sub-Saharan western Africa, J. Hydrol., 370, 52–63, https://doi.org/10.1016/j.jhydrol.2009.02.052, 2009.
Hamilton, S. K., Bunn, S. E., Thoms, M. C., and Marshall, J. C.: Persistence of aquatic refugia between flow pulses in a dryland river system (Cooper Creek, Australia), Limnol. Oceanogr., 50, 743–754, 2005.
Harrington, G. A., Payton Gardner, W., and Munday, T. J.: Tracking Groundwater Discharge to a Large River using Tracers and Geophysics, Groundwater, 52, 837–852, https://doi.org/10.1111/gwat.12124, 2013.
Herczeg, A. L. and Leaney, F. W.: Environmental tracers in arid-zone hydrology, Hydrogeol. J., 19, 17–29, https://doi.org/10.1007/s10040-010-0652-7, 2011.
Huang, J., Chunyu, X., Zhang, D., Chen, X., and Ochoa, C. G.: A framework to assess the impact of eological water conveyance on groundwater-dependent terrestrial ecosystems in arid inland river basins, Sci. Total Environ., 709, 136155, https://doi.org/10.1016/j.scitotenv.2019.136155, 2020.
IPCC: Climate Change 2022: Impacts, Adaptation and Vulnerability. Contribution of Working Group II to the Sixth Assessment Report of the Intergovernmental Panel on Climate Change, edited by: Pörtner, H.-O., Roberts, D. C., Tignor, M., Poloczanska, E. S., Mintenbeck, K., Alegría, A., Craig, M., Langsdorf, S., Löschke, S., Möller, V., Okem, A., and Rama, B., Cambridge University Press. Cambridge University Press, Cambridge, UK and New York, NY, USA, 3056 pp., https://doi.org/10.1017/9781009325844, 2022.
Jackson, C. R. and Pringle, C. M.: Ecological benefits of reduced hydrologic connectivity in intensively developed landscapes, BioScience, 60, 37–46, https://doi.org/10.1525/bio.2010.60.1.8, 2010.
Jaeger, K. and Olden, J.: Electrical resistance sensor arrays as a means to quantify longitudinal connectivity of rivers, River Res. Appl., 28, 1843–1852, https://doi.org/10.1002/rra.1554, 2011.
Jaeger, K. L., Olden, J. D., and Pelland, N. A.: Climate change poised to threaten hydrologic connectivity and endemic fishes in dryland streams, P. Natl. Acad. Sci. USA, 111, 13894–13899, 2014.
Jocque, M., Vanschoenwinkel, B., and Brendonck, L. U. C.: Freshwater rock pools: a review of habitat characteristics, faunal diversity and conservation value, Freshwater Biol., 55, 1587–1602, 2010.
John, K. R.: Survival of Fish in Intermittent Streams of the Chiricahua Mountains, Arizona, Ecology, 45, 112–119, https://doi.org/10.2307/1937112, 1964.
Kalbus, E., Reinstorf, F., and Schirmer, M.: Measuring methods for groundwater – surface water interactions: a review, Hydrol. Earth Syst. Sci., 10, 873–887, https://doi.org/10.5194/hess-10-873-2006, 2006.
Käser, D. H., Binley, A., Heathwaite, A. L., and Krause, S.: Spatio-temporal variations of hyporheic flow in a riffle-step-pool sequence, Hydrol. Process., 23, 2138–2149, https://doi.org/10.1002/hyp.7317, 2009.
Kelso, J. E. and Entrekin, S. A. Intermittent and perennial macroinvertebrate communities had similar richness but differed in species trait composition depending on flow duration, Hydrobiologia, 807, 189–206, 2018.
Knighton, A. D. and Nanson, G. C.: Waterhole form and process in the anastomosing channel system of Cooper Creek, Australia, Geomorphology, 35, 101–117, 2000.
Kresic, N. and Stevanovic, Z.: Groundwater Hydrology of Springs, Elsevier, 592 pp., ISBN 978-1-85617-502-9, 2010.
Labbe, T. R. and Fausch, K. D. Dynamics of intermittent stream habitat regulate persistence of a threatened fish at multiple scales, Ecol. Appl., 10, 1774–1791, https://doi.org/10.1890/1051-0761(2000)010[1774:doishr]2.0.co;2, 2000.
Lamontagne, S, Kirby, J., and Johnston, C.: Groundwater–surface water connectivity in a chain-of-ponds semiarid river, Hydrol. Process., 35, e14129, https://doi.org/10.1002/hyp.14129, 2021.
Lau, J. E., Commander, D. P., and Jacobson, G.: Hydrogeology of Australia. Bureau of Mineral Resources, Geology and Geophysics, Bulletin 227, Australian Government Publishing Service, Canberra, ISBN 0 644 06648 2, 1987.
Lautz, L. K.: Impacts of nonideal field conditions on vertical water velocity estimates from streambed temperature time series, Water Resour. Res., 46, W01509, https://doi.org/10.1029/2009wr007917, 2010.
Leibowitz, S. G. and Brooks, R. T.: Hydrology and landscape connectivity of vernal pools, in: Science and Conservation of Vernal Pools in Northeastern North America, edited by: Calhouh, A. J. K. and deMaynadier, P. G., CRC Press, Boca Raton, FL, USA, 31–53, ISBN 13 978-0-8493-3675-1, 2008.
Leibowitz, S. G., Wigington Jr., P. J., Schofield, K. A., Alexander, L. C., Vanderhoof, M. K., and Golden, H. E.: Connectivity of streams and wetlands to downstream waters: an integrated systems framework, JAWRA J. Am. Water Resour. As., 54, 298–322, https://doi.org/10.1111/1752-1688.12631, 2018.
Leibowitz, S. G., Wigington Jr., P. J., Rains, M. C., and Downing, D. M.: Non-navigable streams and adjacent wetlands: addressing science needs following the Supreme Court's Rapanos decision, Front. Ecol. Environ., 6, 364–371, 2008.
McCallum, J. L. and Shanafield, M.: Residence times of stream-groundwater exchanges due to transient stream stage fluctuations, Water Resour. Res., 52, 2059–2073, https://doi.org/10.1002/2015WR017441, 2016.
McJannet, D., Hawdon, A., Van Neil, T., Boadle, D., Baker, B., Trefry, M., and Rea, I.: Measurement of evaporation from a mine void lake and testing of modelling apporaches, J. Hydrol., 555, 631–647, 2017.
McMahon, T. A., Finlayson, B. L., and Peel, M. C.: Historical development of models for estimating evaporaiton using standard meteorological data, Wires Water, 3, 788–818, 2016.
Manga, M.: On the timescales characterizing groundwater discharge at springs, J. Hydrol., 219, 56–69, 1999.
Marshall, S. K., Cook, P. G., Miller, A. D., Simmons, C. T., and Dogramaci, S.: The effect of undetected barriers on groundwater draw-down and recovery, Groundwater, 57, 718–726, https://doi.org/10.1111/gwat.12856, 2019.
Mather, C. C., Nash, D. J., Dogramaci, S., Grierson, P. F., and Skrzypek, G.: Geomorphic and hydrological controls on groundwater dolocrete formation in the semi-arid Hamersley Basin, northwest Australia, Earth Surf. Proc. Land., 44, 2752–2770, https://doi.org/10.1002/esp.4704, 2019.
Melly, B. L., Schael, D. M., and Gama, P. T.: Perched wetlands: An explanation to wetland formation in semi-arid areas, J. Arid Environ., 141, 34–39, https://doi.org/10.1016/j.jaridenv.2017.02.004, 2017.
Meinzer, O.: Outline of ground-water hydrology with definitions, US Geol. Surv. Water Suppl. Pap., 494, https://doi.org/10.3133/wsp494, 1923.
Meinzer, O. E.: Large springs in the United States, Washington, D.C., Report 557, 119, https://doi.org/10.3133/wsp557, 1927.
Messager, M. L., Lehner, B., Cockburn, C., Lamouroux, N., Pella, H., Snelder, T., Tocknew, K., Trautmann, T., Watt, C., and Datry, T.: Global prevalence of non-perennial rivers and streams, Nature, 594, 391–397, 2021.
Ong, J. B., Lane, J. W., Zlotnik, V. A., Halihan, T., and White, E. A.: Combined use of frequency-domain electromagnetic and electrical resistivity surveys to delineate near-lake groundwater flow in the semi-arid Nebraska Sand Hills, USA, Hydrogeol. J., 18, 1539–1545, https://doi.org/10.1007/s10040-010-0617-x, 2010.
Pearson, M. R., Reid, M. A., Miller, C., and Ryder, D.: Comparison of historical and modern river surveys reveal changes to waterhole characteristics in an Australian dryland river, Geomorphology, 356, 107089, https://doi.org/10.1016/j.geomorph.2020.107089, 2020.
Poeter, E., Fan, Y., Cherry, J., Wood, W., and Mackay, D.: Groundwater in our water cycle – getting to know Earth's most important fresh water source, 136 pp., Groundwater Project, Geulph, Ontario, Canada, ISBN 978-1-7770541-1-3, 2020.
Ponder, W. F.: Mound Springs of the Great Artesian Basin, in: Limnology in Australia, edited by: De Deckker, P. and Williams, W. D., Springer Netherlands, Dordrecht, 403–420, https://doi.org/10.1007/978-94-009-4820-4_25, 1986.
Queensland Government, Queensland. Catchment constrictions, Wetland Info website, https://wetlandinfo.des.qld.gov.au/wetlands/ecology/aquatic-ecosystems-natural/groundwater-dependent/catchment-constrictions/ (last access: 16 June 2021), 2015.
Rau, G. C., Andersen, M. S., McCallum, A. M., and Acworth, R. I.: Analytical methods that use natural heat as a tracer to quantify surface water–groundwater exchange, evaluated using field temperature records, Hydrogeol. J., 18, 1093–1110, https://doi.org/10.1007/s10040-010-0586-0, 2010.
Rau, G. C., Halloran, L. J. S., Cuthbert, M. O., Andersen, M. S., Acworth, R. I., and Tellam, J. H.: Characterising the dynamics of surface water-groundwater interactions in intermittent and ephemeral streams using streambed thermal signatures, Adv. Water Resour., 107, 354–369, https://doi.org/10.1016/j.advwatres.2017.07.005, 2017.
Rayner, T. S., Jenkins, K. M., and Kingsford, R. T.: Small environmental flows, drought and the role of refugia for freshwater fish in the Macquarie Marshes, arid Australia, Ecohydrology: Ecosystems, Land and Water Process Interactions, Ecohydrogeomorphology, 2, 440–453, 2009.
Rhodes, K. A., Proffitt, T., Rowley, T., Knappett, P. S. K., Montiel, D., Dimova, N., Tebo, D., and Miller, G. R.: The importance of bank storage in supplying baseflow to rivers flowing through compartmentalized, alluvial aquifers, Water Resour. Res., 53, 10539–10557, https://doi.org/10.1002/2017WR021619, 2017.
Roshier, D. A., Whetton, P. H., Allan, R. J., and Robertson, A. I.: Distribution and persistence of temporary wetland habitats in arid Australia in relation to climate change, Austral. Ecol., 26, 371–384, 2001.
Scanlon, B. R., Keese, K. E., Flint, A. L., Flint, L. E., Gaye, C. B., Edmunds, M., and Simmers, I.: Global synthesis of groundwater recharge in semiarid and arid regions, Hydrol. Process., 20, 3335–3370, 2006.
Shanafield, M. and Cook, P. G.: Transmission losses, infiltration and groundwater recharge through ephemeral and intermittent streambeds: A review of applied methods, J. Hydrol., 511, 518–529, https://doi.org/10.1016/j.jhydrol.2014.01.068, 2014.
Shanafield, M., Bourke, S. A., Zimmer, M. A., and Costigan, K. H.: An overview of the hydrology of non-perennial rivers and streams, Wiley Interdisciplinary Reviews: Water, 8, e1504, https://doi.org/10.1002/wat2.1504, 2021.
Shannon, J., Richardson, R., and Thornes, J.: Modelling event-based fluxes in ephemeral streams, in: Dryland Rivers: Hydrology and Geomorpohology of Semi-Arid Channels, 129–172, edited by: Bull, L. J. and Kirkby, M. J., John Wiley and Sons, Chichester, England, ISBN 10 9780471491231, ISBN 13 978-0471491231, 2002.
Sharma, D. and Kansal, A.: Assessment of river quality models: a review, Rev. Environ. Sci. Bio, 12, 285–311, https://doi.org/10.1007/s11157-012-9285-8, 2013.
Sheldon, F., Bunn, S. E., Hughes, J. M., Arthington, A. H., Balcombe, S. R., and Fellows, C. S.: Ecological roles and threats to aquatic refugia in arid landscapes: Dryland river waterholes, Mar. Freshw. Res., 61, 885–895, https://doi.org/10.1071/MF09239, 2010.
Shepard, W. D.: Desert springs-both rare and endangered, Aquat. Conserv., 3, 351–359, 1993.
Siebers, A. R., Pettit, N. E., Skrzypek, G., Fellman, J. B., Dogramaci, S., and Grierson, P. F.: Alluvial ground water influences dissolved organic matter biogeochemistry of pools within intermittent dryland streams, Freshwater Biol., 61, 1228–1241, https://doi.org/10.1111/fwb.12656, 2016.
Soti, V., Tran, A., Bailly, J.-S., Puech, C., Seen, D. L., and Bégué, A.: Assessing optical earth observation systems for mapping and monitoring temporary ponds in arid areas, Int. J. Appl. Earth Obs., 11, 344–351, https://doi.org/10.1016/j.jag.2009.05.005, 2009.
Springer, A. E. and Stevens, L. E.: Spheres of discharge of springs, Hydrogeol. J., 17, 83–93, https://doi.org/10.1007/s10040-008-0341-y, 2009.
Stallman, R.: Steady one-dimensional fluid flow in a semi-infinite porous medium with sinusoidal surface temperature, J. Geophys. Res., 70, 2821–2827, 1965.
Stanley, E. H., Fisher, S. G., and Grimm, N. B.: Ecosystem Expansion and Contraction in Streams, BioScience, 47, 427–435, https://doi.org/10.2307/1313058, 1997.
Steward, A. L., von Schiller, D., Tockner, K., Marshall, J. C., and Bunn, S. E.: When the river runs dry: human and ecological values of dry riverbeds, Front. Ecol. Environ., 10, 202–209, 2012.
Stonedahl, S. H., Harvey, J. W., Worman, A., Salehin, M., and Packman, A. I.: A multiscale model for integrating hyporheic exchange from ripples to meanders, Water Resour. Res., 46, W12539, https://doi.org/10.1029/2009wr008865, 2010.
Sturman, A. P. and Tapper, N. J.: The weather and climate of Australia and New Zealand, Oxford University Press, USA, ISBN 0195539230, 1996.
Theis, C. V.: The source of water derived from wells, Civil Envineering, 10, 277–280, 1940.
Thoms, M. C. and Sheldon, F.: Lowland rivers: an Australian introduction, Regul. River., 16, 375–383, 2000.
Townley, L. R. and Trefry, M. G.: Surface water-groundwater interaction near shallow circular lakes: Flow geometry in three dimensions, Water Resour. Res., 36, 935–948, https://doi.org/10.1029/1999wr900304, 2000.
Turner, J. V. and Townley, L. R.: Determination of groundwater flow-through regimes of shallow lakes and wetlands from numerical analysis of stable isotope and chloride tracer distribution patterns, J. Hydrol., 320, 451–483, https://doi.org/10.1016/j.jhydrol.2005.07.050, 2006.
Van der Kamp, G. and Hayashi, M.: Groundwater-wetland ecosystem interaction in the semiarid glaciated plains of North America, Hydrogeol. J., 17, 203–214, 2009.
Villeneuve, S., Cook, P. G., Shanafield, M., Wood, C., and White, N.: Groundwater recharge via infiltration through an ephemeral riverbed, central Australia, J. Arid Environ., 117, 47–58, 2015.
Williams, W. D. and Siebert, B. D.: The chemical composition of some surface waters in central Australia, Mar. Freshwater Res., 14, 166–175, https://doi.org/10.1071/MF9630166, 1963.
Winter, T. C., Harvey, J. W., Franke, O. L., and Alley, W. M.: Groundwater and surface water: a single resource, USGS Circular 1139, https://doi.org/10.3133/cir1139, 1998.
Yu, S.: Ngapa Kunangkul: living water, Report on the Indigenous cultural values of groundwater in the La Grange sub–basin, Perth, Western Australian Water and Rivers Commission, ISBN 0730974782, 2000.
Zlotnik, V. A., Olaguera, F., and Ong, J. B.: An approach to assessment of flow regimes of groundwater-dominated lakes in arid environments, J. Hydrol., 371, 22–30, 2009.
- Abstract
- Introduction
- Hydraulic mechanisms supporting the persistence of instream pools
- Management implications: susceptibility of persistent pools to changing hydrological regimes
- Diagnostic tools for elucidating hydraulic mechanisms supporting pool persistence
- Application of this framework to persistent pools in the Hamersley Basin
- Discussion
- Conclusion
- Data availability
- Author contributions
- Competing interests
- Disclaimer
- Acknowledgements
- Financial support
- Review statement
- References
- Abstract
- Introduction
- Hydraulic mechanisms supporting the persistence of instream pools
- Management implications: susceptibility of persistent pools to changing hydrological regimes
- Diagnostic tools for elucidating hydraulic mechanisms supporting pool persistence
- Application of this framework to persistent pools in the Hamersley Basin
- Discussion
- Conclusion
- Data availability
- Author contributions
- Competing interests
- Disclaimer
- Acknowledgements
- Financial support
- Review statement
- References