the Creative Commons Attribution 4.0 License.
the Creative Commons Attribution 4.0 License.
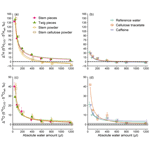
Technical note: On uncertainties in plant water isotopic composition following extraction by cryogenic vacuum distillation
Haoyu Diao
Philipp Schuler
Gregory R. Goldsmith
Rolf T. W. Siegwolf
Matthias Saurer
Recent studies have challenged the interpretation of plant water isotopes obtained through cryogenic vacuum distillation (CVD) based on observations of a large 2H fractionation. These studies have hypothesized the existence of an H-atom exchange between water and organic tissue during CVD extraction with the magnitude of H exchange related to relative water content of the sample; however, clear evidence is lacking. Here, we systematically tested the uncertainties in the isotopic composition of CVD-extracted water by conducting a series of incubation and rehydration experiments using isotopically depleted water, water at natural isotope abundance, woody materials with exchangeable H, and organic materials without exchangeable H (cellulose triacetate and caffeine). We show that the offsets between hydrogen and oxygen isotope ratios and expected reference values (Δ2H and Δ18O) have inversely proportional relationships with the absolute amount of water being extracted, i.e. the lower the water amount, the higher the Δ2H and Δ18O. However, neither Δ2H nor Δ18O values, were related to sample relative water content. The Δ2H pattern was more pronounced for materials with exchangeable H atoms than with non-exchangeable H atoms. This is caused by the combined effect of H exchange during the incubation of materials in water and isotopic enrichments during evaporation and sublimation that depend on absolute water amount. The H exchange during CVD extraction itself was negligible. Despite these technical issues, we observed that the water amount-dependent patterns were much less pronounced for samples at natural isotope abundance and particularly low when sufficiently high amounts of water were extracted (>600 µL). Our study provides new insights into the mechanisms causing isotope fractionation during CVD extraction of water. The methodological uncertainties can be controlled if large samples of natural isotope abundance are used in ecohydrological studies.
- Article
(2436 KB) - Full-text XML
-
Supplement
(476 KB) - BibTeX
- EndNote
Hydrogen and oxygen isotope ratios (δ2H and δ18O) in plant water are powerful tools for tracing the water movement of ecosystems and providing information on the source water used by plants (Goldsmith et al., 2017; Flanagan and Ehleringer, 1991; Brinkmann et al., 2019; Nehemy et al., 2019). The most widely used approach for determining the δ2H and δ18O of plant water is by first extracting water from plants using cryogenic vacuum distillation (CVD) method (Ehleringer et al., 2000; West et al., 2006; Ingraham and Shadel, 1992; Orlowski et al., 2013). Since no isotopic fractionation generally occurs during plant water uptake (White et al., 1985; Zimmermann et al., 1967; Poca et al., 2019), it is generally believed that the isotope ratios in stem water (root crown water for grasses) derived from the CVD extraction (δCVD) are equal to that in the plant source water (δsource). However, an increasing number of studies have found that δCVD, especially , does not actually reflect plant source water, as shown by a strong negative δ2H offset () between CVD-extracted water and source water (Lin and Da S. L. Sternberg, 1993; Zhao et al., 2016; Barbeta et al., 2020; Allen and Kirchner, 2022; Newberry et al., 2017). This issue is important because it may invalidate conclusions on plant water uptake patterns and the partitioning of different pools of water in an ecosystem (Allen et al., 2019; Gessler et al., 2022; Goldsmith et al., 2012; Evaristo and Mcdonnell, 2017).
A critical hypothesis for the observed Δ2H is the spatial heterogeneity of hydrogen isotope composition of water in stems (Zhao et al., 2016; Barbeta et al., 2020), i.e. isotopic differences between stem conductive and nonconductive tissues, and isotopic differences from inside to outside along the stem radial direction. This hypothesis was rejected by Chen et al. (2020). The authors rehydrated dry stem samples of saplings of nine plant species in excess of a reference water with a known isotopic composition for 24 h and then CVD-extracted the water that had soaked into the material. They concluded that the observed negative Δ2H was not caused by within-stem isotope heterogeneity, but was more likely related to a hydrogen atom (H) exchange between stem water and the exchangeable H of stem organic tissue that occurs during the CVD extraction. In woody tissue, oxygen-bound H atoms can exchange with those in surrounding liquid water and water vapour. Theoretically, about 30 % to 50 % of the H atoms of cellulose and its precursors are exchangeable (Schuler et al., 2022; Filot et al., 2006). If the CVD extraction disturbs the previously established isotopic equilibration between organic tissue and tissue water because of the gradually isotopically enriched tissue water during extraction, the potential H exchange would affect ecohydrological interpretations of Δ2H (Barbeta et al., 2020; De La Casa et al., 2022). However, Chen et al. (2020) were unable to isolate the H exchange that occurred during the extraction. This is because their experimental design included two possible H exchange steps: one that occurs during rehydration (hereinafter referred to as “H exchange during rehydration”) and a second one that occurs during the extraction itself (hereinafter referred to as “H exchange during extraction”). Only the latter is of interest, because it is the H exchange process that theoretically affects the isotopic composition of CVD-extracted water from actual plant samples. However, empirical evidence for the occurrence of H exchange and its effects on during rehydration and/or extraction is still lacking.
Moreover, the study of Chen et al. (2020) also found a significant positive correlation between Δ2H and sample relative water content (RWC). They posited that the H exchange effect was probably dampened at high RWC. This correlation has also been found for both Δ2H and Δ18O (i.e. ) in soil samples (Wen et al., 2021). However, using cellulose in wood as an example, much of the O-bound H is engaged in H bonding that links cellulose fibrils together, i.e. the non-freely exchangeable “bridging hydrogen” (Sepall and Mason, 1961; Meier-Augenstein et al., 2014), which requires high temperatures (>100 ∘C) for accessing (Schuler et al., 2022). As such, the freely exchangeable H in cellulose is theoretically only about 5 % (Sepall and Mason, 1961; Meier-Augenstein et al., 2014). If the magnitude of H exchange effect during extraction is therefore moderate, the observed Δ2H would be more readily attributed to isotopic fractionation and mixing that occur after the water is extracted from the sample. If this were to be true, what really matters would not be the RWC, but rather the absolute amount of water being extracted (hereinafter referred to “absolute water amount (AWA)”). The effects of AWA on extraction uncertainties could influence both Δ2H and Δ18O through fractionation during CVD extraction. However, no studies have tested the effect of sample water amount on the CVD extraction biases yet, and the CVD extraction uncertainties during liquid–vapour phase changes are not well known.
During the CVD extraction, successive isotope fractionation processes occur at each phase change of the water (liquid–vapour–solid–liquid). When liquid sample water is distilled to water vapour, isotope fractionation is expected if incomplete extraction occurs (Orlowski et al., 2013; West et al., 2006). When the water vapour is trapped by liquid nitrogen and turns into solid ice, a sublimation isotope fractionation is also expected. Studies testing the sublimation of pure water ice at low temperatures into a vacuum have shown that the sublimated percentage of the bulk water aliquot was <10 % within 1 h at −100 ∘C, and the sublimation rate decreased drastically with the decrease of temperature in the cold trap (Mortimer et al., 2018; Lécuyer et al., 2017). Given the fact that the extracted water is frozen at such a low temperature (−196 ∘C) during CVD extraction, negligible amounts of water should be released into the vapour phase by sublimation during the extraction. Nevertheless, an observable enrichment of the residual ice is still possible as the water lost to the vapour phase is isotopically depleted (Lécuyer et al., 2017; Mortimer et al., 2018). In addition, isotope fractionation related to evaporation and mixing with water vapour in the laboratory probably occur during the end of the CVD extraction (i.e. mixing of the extracted water with air humidity of the lab air when the water traps are removed from the extraction system). Although this can be partly avoided by flushing the extraction line with N2 gas, isotope fractionation can occur when the extracted and frozen water thaws in the water collection tube and when it is transferred to storage vials. A better understanding of these individual isotope fractionation processes during CVD extraction would not only aid in identifying the potential sources of methodological biases (Schoppach and Klaus, 2019) and improving the instrumentation, but also contribute to better estimates of plant water sources by mitigating their effects.
To investigate these open questions, we systematically tested for biases induced by CVD extraction of plant water. A series of experiments that involved extracting water from different kinds of materials (with and without exchangeable H) that were incubated or rehydrated using isotopically depleted water and from plant samples at natural isotope abundance were conducted. Specifically, we hypothesized that the Δ2H is influenced by (i) an H-exchange effect, whereas both Δ2H and Δ18O are influenced by (ii) tissue AWA, and (iii) evaporation and sublimation enrichments. The lower the AWA, the higher the H-exchange effect and evaporation and sublimation fractionation, and the higher the Δ2H and Δ18O.
2.1 Experiment 1: testing the overall H-exchange effect
For evaluating the overall effect of H exchange during both rehydration and extraction on the Δ2H and Δ18O values of CVD-extracted water, we conducted an incubation experiment by adding different amounts of strongly depleted reference water (δ2H: −465.9 ‰; δ18O: −174.2 ‰; hereafter referred to as δref) to the same amount of different dried materials with and without exchangeable H (experiment 1, Fig. 1). The isotopically depleted water was used to make potential isotope effects more evident.
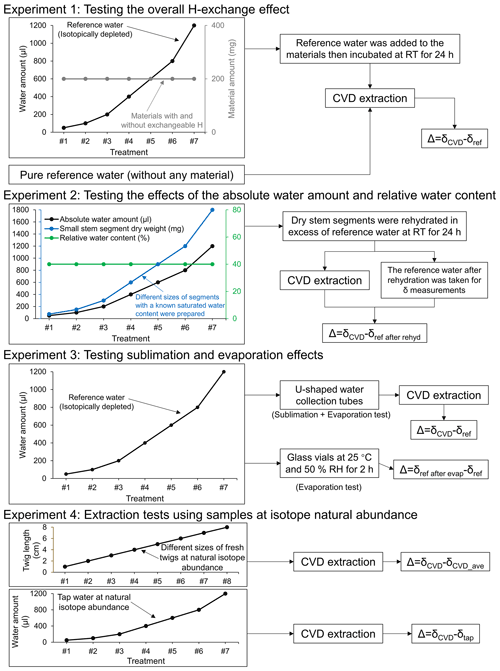
Figure 1Overview of the experimental designs. Δ, isotopic offset; δCVD, isotope composition of CVD-extracted water; δref, isotope composition of the reference water; δref after rehyd, isotope composition of the reference water after rehydration; δref after evap, isotope composition of the reference water after the evaporation; δCVD_ave, the average value of isotope composition of the CVD-extracted water; δtap, isotope composition of the tap water; RT, room temperature; RH, relative humidity.
The materials with exchangeable H included stem pieces, stem powder, purified stem cellulose powder, and twig pieces. The stem materials were obtained from the xylem of a trunk disc of a mature Larix sibirica grown in Siberia (27 cm diameter, 4 cm thickness). We cut a portion of xylem (a mixture of sap wood and heart wood) from the trunk disc and oven dried it to constant weight before preparing for stem pieces, powder, and cellulose materials. Stem pieces were prepared by cutting the xylem of the disc into 4 mm cubes, while the stem powder was prepared by grinding the same material to a homogeneous powder using a steel-ball mill (MM400, Retsch GmbH, Haan, Germany). The stem cellulose was then extracted from ground stem powder (Schuler et al., 2022). The twig pieces were obtained from five young Larix decidua trees growing in a forest in Birmensdorf, Switzerland (47.36∘ N, 8.45∘ E). Two twigs per tree were collected in the morning, outer bark and phloem removed. The twig xylem was oven dried at 60 ∘C for 24 h then cut into 2 mm pieces.
The materials without exchangeable H were cellulose triacetate and caffeine. The cellulose triacetate (C40H54O27; Sigma-Aldrich, St. Louis, MO, USA, prod. no. 22199) is a white granule. The caffeine material is ≥99 % caffeine anhydrous (C8H10N4O2; Fluka Chemie AG, Buchs, Switzerland, prod. no. 27600) in a white crystalline powder form. All H atoms in cellulose triacetate and caffeine are bound to carbon in a form of methyl group (−CH3) and thus non-exchangeable with vapour or liquid water. Cellulose triacetate and caffeine are anhydrous chemicals and were stored in a cool/dry place.
For each material and each different water amount (50, 100, 200, 400, 600, 800, and 1200 µL), three replicates were prepared by transferring 200 mg of each material into 12 mL gas-tight glass vials (Exetainer; Labco, Lampeter, UK). The amount of material was chosen because of practical considerations and to generate a range of water/biomass ratios. The materials were oven dried in the vials with caps open at 60 ∘C for 24 h to remove any remaining moisture in the material. In total, the materials with exchangeable H were oven dried at 60 ∘C for at least 48 h, and the materials without exchangeable H were oven dried at 60 ∘C for 24 h because they are dried chemicals and were properly stored. After drying, the vials were closed and the samples were cooled down to room temperature, then we opened the cap to inject the reference water into the vials. Depending on the form of the material, the low water amounts (e.g. 50 and 100 µL) could not fully wet the material, whereas the high water amounts (e.g. >400 µL) oversaturated the material. Then, the vials were sealed and the materials were incubated for 24 h at room temperature. According to Chen et al. (2020), this incubation time is thought to lead to a full exchange of H atoms between materials and reference water. As a control, the experiment was repeated without any material by adding only the range of reference water into the vial. All samples were frozen at −20 ∘C and then extracted with CVD. Δ was calculated as δCVD−δref.
In addition, to evaluate how effective our drying procedure was and how opening the cap for injecting the reference water influenced the dried samples, we conducted a drying test. In the test, 200 mg stem pieces, stem powder, and caffeine with five replicates were transferred into the vials, then caps closed and weighted. We oven dried the samples without caps for 48 h either at 60 or at 105 ∘C. Every 12 h during the drying, we closed the caps in the oven then transferred the vials to a balance for weighting. After the last weighting, we opened the cap of the vials for 5 s in the lab to simulate the procedure of injecting the reference water. Then the cap closed and samples were weighted again. The percentage of moisture that was removed from the initial sample at different temperatures and hours and the percentage of moisture that was absorbed by the dried sample in the 5 s were calculated (Table S2 in the Supplement).
2.2 Experiment 2: testing the effects of the absolute water amount and relative water content
For separating the H exchange effects on Δ2H and Δ18O values occurring during rehydration from those occurring during extraction, we extracted water from a range of rehydrated stem segments with different sizes (experiment 2, Fig. 1).
Large stem segments ( cm) were cut from the L. sibirica disc (see Sect. 2.1) and oven-dried at 60 ∘C for 24 h before the experiment. We firstly determined the saturated water content of the large stem segments by weighing them before and after they were soaked in excess of deionized water for 24 h. The saturated water content was determined for estimating the AWA of the following smaller stem segment samples. The large stem segments were then oven dried again at 60 ∘C for 24 h and cut into smaller stem segments with different sizes, aiming to generate a range of samples with a narrow RWC (ca. 40 %), but varying in their AWA from 50–1200 µL. The sizes of the small segments were determined based on the proportional relationship between the size and the saturated water content of the large stem segments. Three replicates were produced for each size. Then, the small stem segments were separately soaked in excess of the isotopic depleted reference water (ca. 25 mL) in sealed glass vials at room temperature for 24 h, following Chen et al. (2020). During the 24 h rehydration, the isotope ratios of the original reference water equilibrated with the exchangeable H in the small stem segments. Thus, by the end of the rehydration, the isotope ratios of water in the small stem segments are assumed equal to the isotope ratio of the reference water after rehydration (δref after rehyd) and not to be equal to the original reference water (δref). Subsequently, the small stem segments were taken out of the reference water, the surface water shaken off, and then immediately sealed in vials and frozen at −20 ∘C to prevent evaporation. A sample of the reference water after the rehydration was taken from each tube for determining δref after rehyd. The AWA and the RWC of each small stem segment sample were calculated by comparing the sample weights before and after the extraction. Here, the RWC is defined as , its unit is parts per hundred (%). In experiment 2, Δ was calculated as δCVD−δref after rehyd. This is because it represents the changes in isotope ratios of the water that occurred during the CVD extraction alone.
2.3 Experiment 3: testing sublimation and evaporation effects
For evaluating the potential effects of sublimation and evaporation during CVD extraction on the isotope ratios of the extracted water, different amounts of reference water (50–1200 µL range as above) were added directly into the u-shaped water collection tubes (i.e. in the cold trap) of the CVD extraction system without sample material and then extracted for 2 h following a standard procedure (see Sect. 2.5). The water in the collection tubes was first frozen by the liquid nitrogen cold trap to avoid potential evaporation effects, then the collection tubes were attached to the extraction line for CVD extraction. The experimental design allows us to exclude isotope fractionation related to distillation and condensation occurring when water in the sample vial was relocated to the collection tube (i.e. U-tube), but captures isotope fractionation related to sublimation of the frozen water under the vacuum and evaporation during thawing of water after CVD extraction (experiment 3, Fig. 1). Δ was calculated as δCVD−δref.
For isolating the evaporation effect from the sublimation effect, we also added different amounts of reference water (50–1200 µL range as above) into 2 mL glass vials inside a climate chamber (PGR15, Conviron, Manitoba, Canada). The samples were then left to evaporate with lids open at 25 ∘C and 50 % relative humidity (RH) for 2 h. The samples in the 2 mL glass vials did not require the CVD extraction. The exposed surface area of the water in the 2 mL glass vials was about 64 mm2. Three replicates per water amount were performed for both tests (experiment 3, Fig. 1). Notably, CVD extraction was not used for the evaporation test in the climate chamber, thus the Δ was calculated as the difference between the isotope ratios of the reference water after and before the evaporation (i.e. δref after evap−δref).
2.4 Experiment 4: extraction tests using samples at isotope natural abundance
In order to test whether the CVD-related isotope fractionation, which was observed with labelled water, can also be seen at natural isotope abundance, we performed extractions with tap water and fresh L. decidua twigs with natural isotope abundance to compare with results derived from tests with isotopically depleted reference water. For the tap water (δtap; δ2H: −73.91 ‰, δ18O: −14.43 ‰), the different amounts of water (i.e. 50, 100, 200, 400, 600, 800, and 1200 µL) were added into the vials and kept frozen at −20 ∘C until CVD extraction (experiment 4, Fig. 1). The twigs were collected from five young L. decidua trees growing in a forest in Birmensdorf, Switzerland (see Sect. 2.1). The twig xylem was cut to different lengths (1, 2, 3, 4, 5, 6, 7, and 8 cm) and sealed in vials. The AWA and RWC of each twig sample were determined by weighing before and after CVD extraction. Three replicates were performed per water amount and twig length (experiment 4, Fig. 1). For the extraction with tap water, the Δ was calculated as δCVD−δtap. The isotope ratios of the source water of the L. decidua twigs before extraction were unknown, thus the Δ in this case was calculated as the difference between the δCVD and the average value of δCVD (i.e. δCVD−δCVD_ave).
2.5 Water extraction and stable isotope analysis
We extracted water using a CVD setup similar to the one described by Orlowski et al. (2013). A schematic overview of this setup is shown in Fig. S1 in the Supplement. During extraction, the pressure inside the system was maintained below 0.05 mbar using a vacuum pump. The sample tubes were additionally blocked by PP fiber filters (Nozzle protection filter, Socorex Isba SA, Ecublens, Switzerland) when water was extracted from powder/pieces of the selected materials to avoid particles being drawn into the U-tubes with the extracted water or the vacuum pump (BS2212, Brook Crompton Ltd, Doncaster, UK). The samples in the sample tubes were heated in 80 ∘C water, and the extracted water was condensed and trapped in the collection tubes by liquid nitrogen. The extraction was maintained for 2 h to achieve a complete extraction (West et al., 2006). After the extraction, the vacuum inside the system was released by adding dry nitrogen gas until atmospheric pressure conditions were reestablished. Then the collection tubes with frozen water samples were detached from the system and sealed with rubber plugs. The water in the collection tubes was thawed at room temperature. During this process, the evaporated water vapour usually condensed to form very small water droplets on the inside walls of the collection tube. We consolidated and collected as many of these small water droplets as possible and transferred them into glass vials (350 µL or 2 mL, depending on the extracted water amount; Infochroma AG, Goldau, Switzerland) using a pipette. Syringes and 0.45 µm nylon filters were used if the extracted water appeared to be turbid. The samples were stored at −20 ∘C before and after the extraction.
The δ2H and δ18O of water samples were measured with a high temperature conversion elemental analyser coupled to a DeltaPlus XP isotope ratio mass spectrometer (TC/EA-IRMS; Finnigan MAT, Bermen, Germany). The δ2H and δ18O in the materials without exchangeable H (i.e. cellulose triacetate and caffeine) and δ18O in the bulk organic matter of the materials with exchangeable H were measured with a vario PYRO cube (Elementar Analysensysteme GmbH, Langenselbold, Germany) coupled to a DeltaPlus XP IRMS. Isotope ratios are reported in per mille (‰) relative to Vienna Standard Mean Ocean Water (VSMOW). Calibration versus the international standards was achieved by analysis of a range of certified water of different isotope ratios, resulting in a precision of analyses of 2 ‰ for δ2H and 0.3 ‰ for δ18O. The non-exchangeable δ2H (δ2Hne) of the materials with exchangeable H was determined by pre-treating the materials with a high-temperature water vapour equilibration method according to Schuler et al. (2022). The δ2H and δ18O values of the materials used in the experiments are shown in Table S1 in the Supplement.
2.6 Statistical analyses
To determine if the sample water δ2H and δ18O values were significantly different before and after the CVD extraction, we performed one-sample t-tests for Δ2H and Δ18O values to test if they were significantly different from 0. We fit relationships between Δ2H and Δ18O as a function of AWA using inversely proportional models, and the relationships between Δ2H and Δ18O as a function of RWC using linear models for the best description of the relationships. The relationships between Δ2H and Δ18O as a function of RWC were also tested using a linear mixed-effects model with AWA category (AWA<400 or AWA>400 µL) as a random effect with the lmerTest package (Kuznetsova et al., 2017). All statistics were performed using R version 4.0.4 (R Core Team, 2021).
3.1 H exchange occurs between sample exchangeable H and water during rehydration
Experiment 1 revealed an inversely proportional relationship between the AWA and the Δ2H and Δ18O values of the water derived from material with or without exchangeable H (Fig. 2). In other words, the lower the AWA, the higher the Δ2H and Δ18O values. For both Δ2H and Δ18O, the differences among samples at AWA>600 µL were much smaller compared to those at AWA<600 µL. Noticeably, Δ2H of the water derived from materials with exchangeable H showed a more pronounced pattern, which reached about 150 ‰ at 50 µL AWA, compared to those without exchangeable H (Fig. 2a and b). In contrast, the Δ2H pattern of the water derived from materials without exchangeable H was similar to that of the pure reference water, which reached about 30 ‰ at 50 µL AWA (Fig. 2b). For Δ18O, the patterns were similar among materials with and without exchangeable H, showing an average decrease from 32 ‰ at 50 µL to 1.7 ‰ at 1200 µL (Fig. 2c and d).
The results of experiment 1 suggest that the H exchange during rehydration is mainly responsible for the large Δ2H differences between materials with and without exchangeable H at the low water amounts. This can likely be explained by an isotope mass balance between the exchangeable H in the samples (natural isotope abundance) and H atoms in the reference water (isotopically depleted), with the impact of the exchangeable H of a constant sample size (200 mg) becoming less noticeable as the amount of water increases (from 50 to 1200 µL). Our results thus provide empirical evidence that H exchange occurs during rehydration of material and that this artefact must be considered in rehydration experiments (Chen et al., 2020; Zhao et al., 2022). However, our experiments cannot provide any evidence for the de- and rehydration of plants under natural conditions (i.e. changes in plant relative water content) which can potentially affect isotope composition of plant water (De Deurwaerder et al., 2020; Barbeta et al., 2022). The effect of rehydration under field conditions could be quantified by additional experiments using water with an isotopic composition distinct from that normally expected in soils.
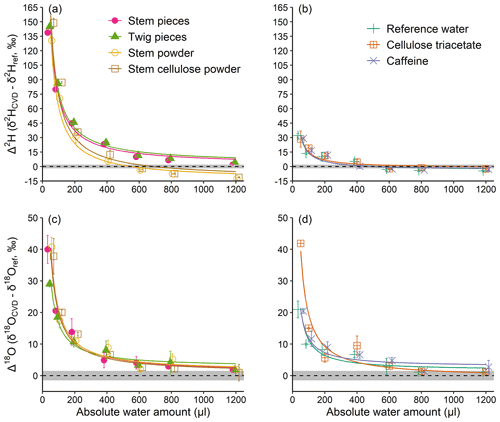
Figure 2Absolute water amount effects on Δ2H and Δ18O values (a, b and c, d, respectively) of water derived from materials with and without exchangeable H (a, c and b, d, respectively), of a constant weight (200 mg). Material with exchangeable H differ in their structural composition (pieces vs. powder). “Reference water” reflects a control performed with water but without any material. (see Sect. 2.1). The dashed lines represent Δ=0, with grey shading to represent the SD of the reference water isotope analyses (2 ‰ for ; 1.5 ‰ for δ18Oref). The curves are inversely proportional fits. Mean values ±1 SD are shown (n=3).
Moreover, the Δ2H of the stem and twig pieces were higher than that of the stem and stem cellulose powder when AWA>600 µL (Fig. 2a), whereas no differences were observed for Δ18O (Fig. 2c). At a first glance, this is unexpected because woody pieces should be less prone to H exchange due to lower surface area and because the OH groups are locked in the matrix of the woody structure (Sepall and Mason, 1961). A possible explanation for the observed Δ2H discrepancies might be an isotopic difference in the exchangeable H of pieces and powdered samples before the start of the experiment. However, if this was true, the effect should be more visible at lower water amounts rather than higher water amounts, as observed in this study. We therefore can conclude that the sample matrix may also influence H exchange in rehydration experiments, but that the underlying mechanisms remain speculative. Besides, we additionally tested whether the drying procedure (oven drying at 60 ∘C) in experiment 1 influenced our results. The test showed that all the tested materials were dried to a constant weight after 36 and 12 h at 60 and 105 ∘C, respectively, despite more moisture being removed at 105 ∘C (Table S2). Interestingly, after opening the cap for 5 s to simulate injection of reference water, the amount of absorbed lab water vapour relative to the dry weight was about 1 % higher for the samples which were dried at 105 ∘C than the samples which were dried at 60 ∘C (Table S2). These results indicate that a complete drying is very difficult in our case, regardless of drying temperature. However, the test provides evidence that only small amounts of remaining moisture remain in the sample after drying and that the reabsorption should not affect the isotopic results of our study, because strongly depleted reference water was used to amplify the isotopic effect.
Unexpectedly, Δ2H values of the pure reference water and of material without exchangeable H, as well as Δ18O values, all show a dependency on AWA (Fig. 2b–d). This observation indicates that Δ values can be biased even when no exchangeable H in plant material is available, and suggests that other factors than H exchange play an important role in biasing isotopic results of CVD-extracted water.
3.2 Absolute water amount not relative water content causes isotope fractionation during CVD extraction
To better understand the unknown isotopic effects during extraction, we performed experiment 2, where we separated the effects between rehydration and extraction on CVD-extracted water along a similar AWA gradient (50–1600 µL), but in a narrow RWC range (36 %–45 %, Fig. 3). The results confirm the inversely proportional pattern of Δ along an AWA gradient as shown by experiment 1 (Fig. 2). More importantly, the results show that Δ values of water extracted from stem material that has been previously rehydrated were similar (Fig. 3a and c) to that extracted from materials without any exchangeable H (Fig. 2b and d), when the isotope ratio of the reference water after rehydration (δref after rehyd) instead of the reference water was considered. This shows that the H-exchange effect during extraction (i.e. all steps after the rehydration procedure) is negligible and cannot be the main reason for observed isotopic offset between expected and measured isotope ratios in water of stem material (Bowers and Williams, 2022).
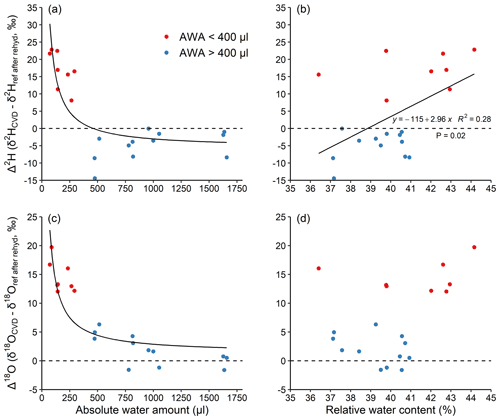
Figure 3Effects of absolute water amount and relative water content (a, c and b, d, respectively) on Δ2H and Δ18O of water derived from the stem segments (a, b and c, d, respectively). The stem segments were rehydrated in excess of the reference water followed by a CVD extraction. (see Sect. 2.2). The relationship between AWA and Δ was fit with an inversely proportional function; the relationship between RWC and Δ was fit with a linear regression. The dashed lines represent Δ=0. Different colours were used to visually separate data with water amount <400 (red) and >400 µL (blue).
This finding is in contrast to the conclusions made by Chen et al. (2020), stating that stem water CVD extraction error could originate from a dynamic H exchange between sample and water during extraction. The reason for the lack of H-exchange effect during the 2 h CVD extraction itself is probably because the duration for the H exchange was too short to fully exchange. Previous research shows that a large portion of the water in the sample is expected to be extracted within the first 30 min of extraction (Orlowski et al., 2013; West et al., 2006). Thus, there may not be a strong H exchange occurring between the water and sample tissue, especially when a large portion of exchangeable H atoms are not freely accessible at the extraction temperature of 80 ∘C (Sepall and Mason, 1961). The duration of rehydration might be another factor that potentially affected our results of the rehydration experiments (experiment 1 and 2). According to Chen et al. (2020), a complete equilibration between sample exchangeable H and reference water during rehydration can be achieved within 24 h, given water of a sufficiently large volume. In experiment 2 of our study, the rehydration was also conducted in an excess of reference water for 24 h. The AWA-dependence of Δ2H values of stem segments in experiment 2 (Fig. 3a) was found to be similar to that of the pure reference water and materials without exchangeable H extractions in experiment 1 (Fig. 2b). This likely confirmed that a full equilibrium was achieved during our 24 h rehydration. Nevertheless, the duration, sample size, and amount of water should be considered in future rehydration studies.
We found a weak, positive linear trend in Δ2H with RWC (r2=0.28, p=0.02, Fig. 3b), but not for Δ18O (p=0.08, Fig. 3d). The trend in Δ2H can be explained by the fact that samples with a smaller AWA (<400 µL) had a ca. 2 % higher RWC compared to those with a higher AWA (>400 µL). The relationships between both Δ2H and Δ18O as a function of RWC were not statistically significant when AWA was considered as a random factor (AWA with 2 levels: AWA<400 and AWA>400 µL; Fig. 3 and Table S3 in the Supplement). This indicates that the Δ2H and Δ18O values are dependent on AWA rather than on RWC of the sample. Our result is not consistent with Chen et al. (2020), who found a significant positive correlation of stem RWC with the Δ2H in a rehydration experiment and further recommended determining sample RWC for correcting CVD artefacts. However, our results are well supported by the recent study of Zhao et al. (2022), who CVD-extracted water from rehydrated samples of 12 woody plant species and analysed Δ values. The RWC of their samples ranged from 30 %–60 % and no significant relationships were found between both Δ2H and Δ18O and RWC. Therefore, the relatively smaller sample RWC range in our study (ca. 10 %) compared to that in Chen et al. (2020), i.e. ca. 20 % for most samples, could not be the reason for the inconsistency of the two studies. Further, given that AWA is typically not reported, we could not test whether the AWA or RWC caused the effect in previous studies. We highlight that the AWA rather than the RWC of a sample should be considered to potentially correct for Δ offsets, and that future studies should report AWA in order to quantify the CVD-induced isotopic biases across laboratories.
3.3 On the processes causing isotope fractionation during CVD extraction
In order to better understand the processes leading to isotope fractionation during CVD, we performed an additional experiment (experiment 3). In this experiment, different amounts of reference water were either injected directly into the collection tubes then subject to CVD extraction, or subject to controlled evaporative conditions in a climate chamber for 2 h. Interestingly, experiment 3 shows that both Δ2H and Δ18O values of reference water, that was directly transferred to the cold trap (i.e. U-tubes), also followed the inversely proportional pattern as a function of AWA (Fig. 4a). At the lowest AWA, Δ2H reached about 19 ‰, while Δ18O reached about 9 ‰. For AWA>600 µL, the average Δ2H and Δ18O were −1.5 ‰ and −2.3 ‰, respectively, and both were significantly different from 0 ( and −3.34, df=8, p<0.01). We suppose that the negative Δ2H and Δ18O values for the AWA>600 µL were purely caused by the analytical uncertainty, because no incomplete extraction could occur given that the reference water was added directly into the collection tube. The same inversely proportional pattern was observed when water was evaporated under controlled conditions in a climate chamber, whereby Δ2H reached about 46 ‰ and Δ18O reached about 23 ‰ at the lowest AWA. For AWA>600 µL, average Δ2H was about 3 ‰ and significantly different from 0 (t=12.15, df=8, p<0.01). No significant difference from 0 was found for Δ18O (t=0.23, df=8, p=0.8). By comparing these results with the one of 50 µL of reference water that was extracted from the sample tube (Fig. 2b), the sublimation and evaporation isotope fractionation account for 59 % and 38 % of the observed Δ2H and Δ18O, respectively. The unaccounted isotope fractionation may originate from distillation and condensation, when water in the sample tube was relocated to the water collection tube.
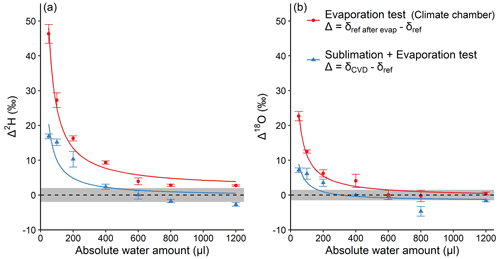
Figure 4Absolute water amount effects on Δ2H (a) and Δ18O (b) of the reference water that was directly added into the water collection tube before CVD extraction (blue), or evaporated in a climate chamber (red). For the former, ; for the latter, (see Sect. 2.3). The dashed lines represent Δ=0, with grey shading to represent the SD of the reference water isotope analyses (2 ‰ for ; 1.5 ‰ for δ18Oref). The curves are inversely proportional fits. Mean values ±1 SD are shown (n=3).
Two potential reasons can explain this water amount-dependent pattern of Δ. On the one hand, a bi-directional exchange between water droplets and water vapour may lead to an isotopic equilibration between the two sources, similar to observations at the leaf level (Lehmann et al., 2020; Goldsmith et al., 2017). This process is expected to occur when the extracted water drop was thawing inside the water collection tube after extraction. The equilibration effect was presumably large on our extracted water, because of a high isotopic discrepancy between the water vapour of the laboratory (at natural isotope abundance) and the isotopically depleted reference water. The effect is expected to increase as the water pool size decreases, because the smaller the water droplets, the larger the ratio of water vapour volume to water droplets volume in the water collection tube. Therefore, isotope ratios of smaller water amounts were subject to greater influence of the isotopic composition of the laboratory water vapour. On the other hand, the sublimation, evaporation, and/or exchange with surrounding water vapour occurs at the surface of water drops (Stewart, 1975). The smaller the water drop, the larger the ratio of the enriched surface water to the total water drop volume. Thus, the mixing effect of enriched surface water and the rest of the water body would be greater for smaller water drops.
Taken together, our results clearly show that the inversely proportional pattern of Δ along AWA is partly influenced by the isotope fractionation during water sublimation and evaporation during CVD extraction. We therefore conclude that isotope fractionation rather than H-exchange effects with plant material during extraction itself should be considered as factors during CVD extraction of plant water. It should be noted that although our experiments were mainly conducted on samples of Larix, the AWA-dependent patterns of Δ were consistent among extractions with different materials and even pure water. This indicates that the CVD biases we observed on Larix may also be applicable to other taxa. The magnitude of the biases may be slightly different among species (Zhao et al., 2022; Chen et al., 2020; De La Casa et al., 2022), but the AWA-dependency of the biases should show consistency. We also want to highlight that the observed isotope fractionation likely depends on the setup (e.g. system volume) of the CVD extraction system. Therefore, further laboratory comparisons for plant water extraction using different woody and herbaceous plant species are needed for constraining this extraction bias.
3.4 Relevance for isotope fractionation during CVD extraction for samples at natural isotope abundance
The large isotopic discrepancy between the strongly depleted reference water and the plant tissue organic matter allowed us to investigate the processes and mechanisms driving isotope fractionation during CVD extraction. However, plant water at natural isotope abundance is typically more enriched compared to our reference water (δ2H: −465.9 ‰; δ18O: −174.2 ‰) and therefore the observed isotopic effects of this study are likely less pronounced at natural isotope abundance. This was true, as shown by the results of experiment 4; the inversely proportional pattern was less pronounced or absent with plant samples (average values in δ2H and δ18O of extracted water: −58.16 ‰ and −7.84 ‰, respectively), or when tap water (δ2H: −73.91 ‰; δ18O: −14.43 ‰) was used (Fig. 5). For the pure tap water extraction, Δ2H decreased from 3 ‰ at 50 µL to −3.5 ‰ at 1200 µL, while Δ18O decreased from 2 ‰ at 50 µL to −0.2 ‰ at 1200 µL (Fig. 5a and c).
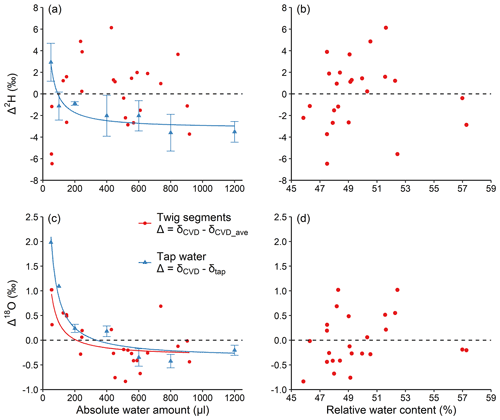
Figure 5Absolute water amount and relative water content effects (a, c and b, d, respectively) on Δ2H and Δ18O (a, b and c, d, respectively) of water at natural isotope abundance. For water of Larix decidua twig xylem, ; while for the tap water, (see Sect. 2.4). The relationship between water amount and Δ was fit with an inversely proportional function. The dashed lines represent Δ=0. For (a) and (c), mean values ±1 SD are shown (n=3).
The possible explanations for the large difference in the magnitude of the Δ pattern between the reference (Fig. 2b and d) and tap water extractions (Fig. 5a and c) are that the isotope fractionation during CVD extraction is dependent on the initial water isotope ratios and/or the influence from isotope ratios of the surrounding water vapour. Regarding the former, water evaporation tests using water with different isotope ratios (δ2H range: −14.7 ‰ to −57.5 ‰; δ18O range: 3.4 ‰ to −7.8 ‰) showed that the δ2H and δ18O values of the initial water have little influence on water evaporation isotope fractionation conducted by Hu et al. (2009). In other words, for water with different initial isotope ratios evaporating in the same conditions, the changes in δ2H or δ18O would be almost the same. Therefore, the initial water isotope ratios would not be the reason for the significant Δ difference between the reference water and the tap water extraction.
It is therefore possible that the difference in Δ between reference water and tap water extractions is caused by the exchange of extracted water with the water vapour in the laboratory. The isotope ratios of the laboratory water vapour are closer to that of the tap water and twig water, but differ greatly compared to the depleted reference water. Therefore, under these conditions, the initial isotopic signature of the extracted water determines the magnitude of the isotope signature of the extracted water after the exchange with laboratory water vapour and therefore the observed Δ values. This suggests that the observed isotopic variations in our experiments with strongly isotopically depleted reference water might have been less pronounced if water closer to natural isotope abundance would have been used.
For the fresh L. decidua twigs water extraction, the AWA-dependent pattern was evident for Δ18O, with average values decreasing from approximately 1 ‰ at the lowest water amount to approximately −0.2 ‰ at the highest water amount (Fig. 5c). In contrast, Δ2H ranged between −6.5 ‰ and 6.1 ‰ but without showing a clear inversely proportional pattern (Fig. 5a). While we found no clear explanation for the absence of the AWA dependency for Δ2H in this experiment, we can only speculate that the expected pattern was hidden by processes shaping hydrogen but not oxygen isotopic variations such as H-exchange effects between water vapour and extracted water with similar isotopic compositions. Our results also showed that the Δ values obtained from the extraction of water at natural isotope abundance (Fig. 5a and c) are much smaller compared to those obtained from the extractions of the strongly depleted reference water (Figs. 2 and 3). In addition, we did not find a significant correlation between RWC and Δ2H or Δ18O for the L. decidua twigs at a RWC range between 45 % and 57 % (Fig. 5b and d). These results suggest again that the Δ2H and Δ18O of the CVD-extracted water is dependent on AWA rather than on RWC.
In conclusion, we provide strong evidence that H exchange with organic H in the sample, despite significantly influencing the δ2H of water during the sample rehydration process, is not the main driver of the broadly observed negative Δ2H value during CVD extraction. Instead, we identified a significant CVD artefact when water is present in small amounts, particularly when δ2H and δ18O of the water were below natural isotope abundance. This is linked to the increase of sublimation and evaporation enrichments with the decrease of water amount, as well as the mixing between the extracted water and laboratory water vapour, rather than the effect of sample RWC on the H exchange during extraction. From our results, both Δ2H and Δ18O approached steady values and were close to zero when >600 µL of water was extracted. We therefore recommend extracting more than 600 µL of water, especially for studies using labelled water, to avoid large enrichment biases. However, the minimum amount of extracted water that is necessary to avoid isotopic biases might vary with the CVD setup and therefore laboratory comparison should be conducted. Our results have implications for studies using stable isotopes of water in plant tissue to determine plant water sources (e.g. Evaristo and McDonnell, 2017), trace water through soils with high organic material content (e.g. Koeniger et al., 2016; Sprenger et al., 2016), and reconstruct climate patterns using tree ring tissue (e.g. Loader et al., 2007; Lehmann et al., 2021).
The code used for all statistical analyses is available upon request.
Data are available from the corresponding author upon reasonable request.
The supplement related to this article is available online at: https://doi.org/10.5194/hess-26-5835-2022-supplement.
HD, PS, GRG, and MML designed the experiments and HD carried them out. HD prepared the paper with contributions from all co-authors.
The contact author has declared that none of the authors has any competing interests.
Publisher's note: Copernicus Publications remains neutral with regard to jurisdictional claims in published maps and institutional affiliations.
We acknowledge the technical assistance by Manuela Oettli and Oliver Rehmann at WSL. Haoyu Diao acknowledges a scholarship from the Joint PhD Training Program, University of Chinese Academy of Sciences.
This research has been supported by the SNSF Ambizione project “TreeCarbo” (grant no. 179978, granted to Marco M. Lehmann) and the USDA-NIFA (grant no. 2020-67014-30917, granted to Gregory R. Goldsmith).
This paper was edited by Markus Weiler and reviewed by two anonymous referees.
Allen, S. T. and Kirchner, J. W.: Potential effects of cryogenic extraction biases on plant water source partitioning inferred from xylem water isotope ratios, Hydrol. Process., 36, e14483, https://doi.org/10.1002/hyp.14483, 2022.
Allen, S. T., Kirchner, J. W., Braun, S., Siegwolf, R. T. W., and Goldsmith, G. R.: Seasonal origins of soil water used by trees, Hydrol. Earth Syst. Sci., 23, 1199–1210, https://doi.org/10.5194/hess-23-1199-2019, 2019.
Barbeta, A., Gimeno, T. E., Clavé, L., Fréjaville, B., Jones, S. P., Delvigne, C., Wingate, L., and Ogée, J.: An explanation for the isotopic offset between soil and stem water in a temperate tree species, New Phytol., 227, 766–779, https://doi.org/10.1111/nph.16564, 2020.
Barbeta, A., Burlett, R., Martín-Gómez, P., Fréjaville, B., Devert, N., Wingate, L., Domec, J.-C., and Ogée, J.: Evidence for distinct isotopic compositions of sap and tissue water in tree stems: consequences for plant water source identification, New Phytol., 233, 1121–1132, https://doi.org/10.1111/nph.17857, 2022.
Bowers, W. H. and Williams, D. G.: Isotopic Heterogeneity of Stem Water in Conifers Is Correlated to Xylem Hydraulic Traits and Supports Multiple Residence Times, Frontiers in Water, 4, 861590, https://doi.org/10.3389/frwa.2022.861590, 2022.
Brinkmann, N., Eugster, W., Buchmann, N., and Kahmen, A.: Species-specific differences in water uptake depth of mature temperate trees vary with water availability in the soil, Plant Biol., 21, 71–81, https://doi.org/10.1111/plb.12907, 2019.
Chen, Y., Helliker, B. R., Tang, X., Li, F., Zhou, Y., and Song, X.: Stem water cryogenic extraction biases estimation in deuterium isotope composition of plant source water, P. Natl. Acad. Sci. USA, 117, 33345–33350, https://doi.org/10.1073/pnas.2014422117, 2020.
De Deurwaerder, H. P. T., Visser, M. D., Detto, M., Boeckx, P., Meunier, F., Kuehnhammer, K., Magh, R.-K., Marshall, J. D., Wang, L., Zhao, L., and Verbeeck, H.: Causes and consequences of pronounced variation in the isotope composition of plant xylem water, Biogeosciences, 17, 4853–4870, https://doi.org/10.5194/bg-17-4853-2020, 2020.
de la Casa, J., Barbeta, A., Rodríguez-Uña, A., Wingate, L., Ogée, J., and Gimeno, T. E.: Isotopic offsets between bulk plant water and its sources are larger in cool and wet environments, Hydrol. Earth Syst. Sci., 26, 4125–4146, https://doi.org/10.5194/hess-26-4125-2022, 2022.
Ehleringer, J. R., Roden, J., and Dawson, T. E.: Assessing ecosystem-level water relations through stable isotope ratio analyses, in: Methods in ecosystem science, edited by: Sala, O. E., Jackson, R. B., Mooney, A. A., and Howarth, R. W., Springer, 181–198, https://doi.org/10.1007/978-1-4612-1224-9_13, 2000.
Evaristo, J. and McDonnell, J. J.: Prevalence and magnitude of groundwater use by vegetation: a global stable isotope meta-analysis, Sci. Rep.-UK, 7, 44110, https://doi.org/10.1038/srep44110, 2017.
Filot, M. S., Leuenberger, M., Pazdur, A., and Boettger, T.: Rapid online equilibration method to determine the ratios of non-exchangeable hydrogen in cellulose, Rapid Commun. Mass Sp., 20, 3337–3344, https://doi.org/10.1002/rcm.2743, 2006.
Flanagan, L. B. and Ehleringer, J. R.: Stable Isotope Composition of Stem and Leaf Water: Applications to the Study of Plant Water Use, Funct. Ecol., 5, 270–277, https://doi.org/10.2307/2389264, 1991.
Gessler, A., Bächli, L., Rouholahnejad Freund, E., Treydte, K., Schaub, M., Haeni, M., Weiler, M., Seeger, S., Marshall, J., Hug, C., Zweifel, R., Hagedorn, F., Rigling, A., Saurer, M., and Meusburger, K.: Drought reduces water uptake in beech from the drying topsoil, but no compensatory uptake occurs from deeper soil layers, New Phytol., 233, 194–206, https://doi.org/10.1111/nph.17767, 2022.
Goldsmith, G. R., Muñoz-Villers, L. E., Holwerda, F., McDonnell, J. J., Asbjornsen, H., and Dawson, T. E.: Stable isotopes reveal linkages among ecohydrological processes in a seasonally dry tropical montane cloud forest, Ecohydrology, 5, 779–790, https://doi.org/10.1002/eco.268, 2012.
Goldsmith, G. R., Lehmann, M. M., Cernusak, L. A., Arend, M., and Siegwolf, R. T. W.: Inferring foliar water uptake using stable isotopes of water, Oecologia, 184, 763–766, https://doi.org/10.1007/s00442-017-3917-1, 2017.
Hu, H.-Y., Bao, W.-M., Wang, T., and Qu, S.-M.: Experimental study on stable isotopic fractionation of evaporating water under varying temperature, Water Sci. Eng., 2, 11–18, 2009.
Ingraham, N. L. and Shadel, C.: A comparison of the toluene distillation and vacuum/heat methods for extracting soil water for stable isotopic analysis, J. Hydrol., 140, 371–387, https://doi.org/10.1016/0022-1694(92)90249-U, 1992.
Koeniger, P., Gaj, M., Beyer, M., and Himmelsbach, T.: Review on soil water isotope-based groundwater recharge estimations, Hydrol. Process., 30, 2817–2834, https://doi.org/10.1002/hyp.10775, 2016.
Kuznetsova, A., Brockhoff, P. B., and Christensen, R. H. B.: lmerTest Package: Tests in Linear Mixed Effects Models, J. Stat. Softw., 82, 1–26, https://doi.org/10.18637/jss.v082.i13, 2017.
Lécuyer, C., Royer, A., Fourel, F., Seris, M., Simon, L., and Robert, F.: fractionation during the sublimation of water ice, Icarus, 285, 1–7, https://doi.org/10.1016/j.icarus.2016.12.015, 2017.
Lehmann, M. M., Goldsmith, G. R., Mirande-Ney, C., Weigt, R. B., Schönbeck, L., Kahmen, A., Gessler, A., Siegwolf, R. T. W., and Saurer, M.: The 18O-signal transfer from water vapour to leaf water and assimilates varies among plant species and growth forms, Plant Cell Environ., 43, 510–523, https://doi.org/10.1111/pce.13682, 2020.
Lehmann, M. M., Vitali, V., Schuler, P., Leuenberger, M., and Saurer, M.: More than climate: Hydrogen isotope ratios in tree rings as novel plant physiological indicator for stress conditions, Dendrochronologia, 65, 125788, https://doi.org/10.1016/j.dendro.2020.125788, 2021.
Lin, G. and da S. L. Sternberg, L.: 31 – Hydrogen Isotopic Fractionation by Plant Roots during Water Uptake in Coastal Wetland Plants, in: Stable Isotopes and Plant Carbon-water Relations, edited by: Ehleringer, J. R., Hall, A. E., and Farquhar, G. D., Academic Press, San Diego, 497–510, https://doi.org/10.1016/B978-0-08-091801-3.50041-6, 1993.
Loader, N. J., McCarroll, D., Gagen, M., Robertson, I., and Jalkanen, R.: Extracting climatic information from stable isotopes in tree rings, in: Terrestrial Ecology, edited by: Dawson, T. E. and Siegwolf, R. T. W., Elsevier, 25–48, https://doi.org/10.1016/S1936-7961(07)01003-2, 2007.
Meier-Augenstein, W., Kemp, H. F., Schenk, E. R., and Almirall, J. R.: Discrimination of unprocessed cotton on the basis of geographic origin using multi-element stable isotope signatures, Rapid Commun. Mass Sp., 28, 545–552, https://doi.org/10.1002/rcm.6811, 2014.
Mortimer, J., Lécuyer, C., Fourel, F., and Carpenter, J.: fractionation during sublimation of water ice at low temperatures into a vacuum, Planet. Space Sci., 158, 25–33, https://doi.org/10.1016/j.pss.2018.05.010, 2018.
Nehemy, M. F., Benettin, P., Asadollahi, M., Pratt, D., Rinaldo, A., and McDonnell, J. J.: How plant water status drives tree source water partitioning, Hydrol. Earth Syst. Sci. Discuss. [preprint], https://doi.org/10.5194/hess-2019-528, 2019.
Newberry, S. L., Nelson, D. B., and Kahmen, A.: Cryogenic vacuum artifacts do not affect plant water-uptake studies using stable isotope analysis, Ecohydrology, 10, e1892, https://doi.org/10.1002/eco.1892, 2017.
Orlowski, N., Frede, H.-G., Brüggemann, N., and Breuer, L.: Validation and application of a cryogenic vacuum extraction system for soil and plant water extraction for isotope analysis, J. Sens. Sens. Syst., 2, 179–193, https://doi.org/10.5194/jsss-2-179-2013, 2013.
Poca, M., Coomans, O., Urcelay, C., Zeballos, S. R., Bodé, S., and Boeckx, P.: Isotope fractionation during root water uptake by Acacia caven is enhanced by arbuscular mycorrhizas, Plant Soil, 441, 485–497, https://doi.org/10.1007/s11104-019-04139-1, 2019.
R Core Team: R: A Language and Environment for Statistical Computing, R Foundation for Statistical Computing, https://www.R-project.org/ (last access: 15 November 2022), 2021.
Schoppach, R. and Klaus, J.: Is cryogenic vacuum distillation reliable for wood water extraction?, EGU General Assembly 2019, 7–12 April 2019, Vienna, Austria, 4901, 2019.
Schuler, P., Cormier, M.-A., Werner, R. A., Buchmann, N., Gessler, A., Vitali, V., Saurer, M., and Lehmann, M. M.: A high-temperature water vapor equilibration method to determine non-exchangeable hydrogen isotope ratios of sugar, starch and cellulose, Plant Cell Environ., 45, 12–22, https://doi.org/10.1111/pce.14193, 2022.
Sepall, O. and Mason, S. G.: Hydrogen exchange between cellulose and water: II. Interconversion of accessible and inaccessible regions, Can. J. Chem., 39, 1944–1955, https://doi.org/10.1139/v61-261, 1961.
Sprenger, M., Leistert, H., Gimbel, K., and Weiler, M.: Illuminating hydrological processes at the soil-vegetation-atmosphere interface with water stable isotopes, Rev. Geophys., 54, 674–704, https://doi.org/10.1002/2015RG000515, 2016.
Stewart, M. K.: Stable isotope fractionation due to evaporation and isotopic exchange of falling waterdrops: Applications to atmospheric processes and evaporation of lakes, J. Geophys. Res., 80, 1133–1146, https://doi.org/10.1029/JC080i009p01133, 1975.
Wen, M., Lu, Y., Li, M., He, D., Xiang, W., Zhao, Y., Cui, B., and Si, B.: Correction of cryogenic vacuum extraction biases and potential effects on soil water isotopes application, J. Hydrol., 603, 127011, https://doi.org/10.1016/j.jhydrol.2021.127011, 2021.
West, A. G., Patrickson, S. J., and Ehleringer, J. R.: Water extraction times for plant and soil materials used in stable isotope analysis, Rapid Commun. Mass Sp., 20, 1317–1321, https://doi.org/10.1002/rcm.2456, 2006.
White, J. W. C., Cook, E. R., Lawrence, J. R., and Wallace S, B.: The DH ratios of sap in trees: Implications for water sources and tree ring DH ratios, Geochim. Cosmochim. Ac., 49, 237–246, https://doi.org/10.1016/0016-7037(85)90207-8, 1985.
Zhao, L., Wang, L., Cernusak, L. A., Liu, X., Xiao, H., Zhou, M., and Zhang, S.: Significant difference in hydrogen isotope composition between xylem and tissue water in Populus Euphratica, Plant Cell Environ., 39, 1848–1857, https://doi.org/10.1111/pce.12753, 2016.
Zhao, P., Sprenger, M., Barzegar, R., Tang, X., and Adamowski, J.: Similar isotopic biases of plant stems bulk water from different water sources by cryogenic vacuum distillation demonstrated through rehydration experiments, Geophys. Res. Lett., 49, e2021GL096474, https://doi.org/10.1029/2021GL096474, 2022.
Zimmermann, U., Ehhalt, D., and Muennich, K. O.: Soil-water movement and evapotranspiration: Changes in the isotopic composition of the water, Isotopes in Hydrology, 567–585, https://inis.iaea.org/search/searchsinglerecord.aspx?recordsFor=SingleRecord&RN=38061083 (last access: 15 November 2022), 1967.