the Creative Commons Attribution 4.0 License.
the Creative Commons Attribution 4.0 License.
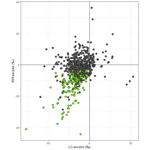
Isotopic offsets between bulk plant water and its sources are larger in cool and wet environments
Javier de la Casa
Adrià Barbeta
Asun Rodríguez-Uña
Lisa Wingate
Jérôme Ogée
Teresa E. Gimeno
Isotope-based approaches to study plant water sources rely on the assumption that root water uptake and within-plant water transport are non-fractionating processes. However, a growing number of studies have reported offsets between plant and source water stable isotope composition for a wide range of ecosystems. These isotopic offsets can result in the erroneous attribution of source water used by plants and potential overestimations of groundwater uptake by the vegetation. We conducted a global meta-analysis to quantify the magnitude of these plant source water isotopic offsets and explored whether their variability could be explained by either biotic or abiotic factors. Our database compiled 112 studies spanning arctic to tropical biomes that reported the dual water isotope composition (δ2H and δ18O) of plant (stem) and source water, including soil water (sampled following various methodologies and along a variable range of depths). We calculated plant source 2H offsets in two ways: a line conditioned excess (LC-excess) that describes the 2H deviation from the local meteoric water line and a soil water line conditioned excess (SW-excess) that describes the deviation from the soil water line, for each sampling campaign within each study. We tested for the effects of climate (air temperature and soil water content), soil class, and plant traits (growth form, leaf habit, wood density, and parenchyma fraction and mycorrhizal habit) on LC-excess and SW-excess. Globally, stem water was more depleted in 2H than in soil water (SW-excess < 0) by 3.02±0.65 ‰ (P < 0.05 according to estimates of our linear mixed model and weighted by sample size within studies). In 95 % of the cases where SW-excess was negative, LC-excess was negative, indicating that the uptake of water that had not undergone evaporative enrichment (such as groundwater) was unlikely to explain the observed soil–plant water isotopic offsets. Soil class and plant traits did not have any significant effect on SW-excess. SW-excess was more negative in cold and wet sites, whereas it was more positive in warm sites. The climatic effects on SW-excess suggest that methodological artefacts are unlikely to be the sole cause of observed isotopic offsets. Our results would imply that plant source water isotopic offsets may lead to inaccuracies when using the isotopic composition of bulk stem water as a proxy to infer plant water sources.
- Article
(550 KB) - Full-text XML
-
Supplement
(107 KB) - BibTeX
- EndNote
For decades, it has been suggested that the stable isotope composition of water (i.e. its 2H 1H and 18O 16O ratios, usually reported in ‰ VSMOW as δ2H and δ18O, respectively) in plant stems could be used to identify the origin of root water uptake and plant transpiration (Ehleringer and Dawson, 1992). A comparison of the isotopic composition of plant water with that of its potential sources has served to infer groundwater uptake in arid and semi-arid environments (e.g. Illuminati et al., 2022; Thorburn et al., 1995; Yin et al., 2015), characterize seasonal shifts in root water uptake across the soil profile (Eggemeyer et al., 2009; Schwendenmann et al., 2015), or unveil the use of alternative water sources such as dew or fog (Burgess and Dawson, 2004). In the past decade, methodological advances, such as novel statistical tools (Stock et al., 2018) and high throughput of samples using laser-based instruments (Martín-Gómez et al., 2015), have allowed for significant increases in the spatio-temporal resolution of water isotope data sets that can be used to infer plant water sources. Recently, several meta-analyses have compiled these studies and found that water stored in the unsaturated zone is likely the main water source accessed by vegetation (Amin et al., 2020), with notable exceptions in arid and semi-arid environments where groundwater forms a significant contribution to the plant water budget (Barbeta and Peñuelas, 2017; Evaristo and McDonnell, 2017).
The attribution of plant water sources from the analysis of water stable isotope composition relies heavily on the assumption that the isotopic composition of plant stem water reflects that of its source. This is because root water uptake is generally considered a non-fractionating process, so that plant and source water should have the same isotopic composition (Ehleringer and Dawson, 1992). This lack of fractionation was supported experimentally more than 80 years ago for plants grown hydroponically (Washburn and Smith, 1934; Zimmermann et al., 1967), and ever since, numerous published field studies have reported plant water isotope compositions that correspond well to a mixture of ecologically relevant potential water sources (e.g. Brunel et al., 1997; Liu et al., 2019; Rong et al., 2011; Schwendenmann et al., 2015). However, it was also shown that isotopic offsets between plant and soil water could be found in some plants adapted to growing in xeric and saline environments (Ellsworth and Williams, 2007; Lin and Sternberg, 1993). More recently, an isotopic offset between plant stem water and pot soil water has been identified in various glasshouse experiments with non-halophytic and non-xerophytic plant species (Vargas et al., 2017; Barbeta et al., 2020). In addition, another recent glasshouse study showed that this isotopic offset was larger in plants forming symbiotic associations with mycorrhizal fungi (Poca et al., 2019). Previous studies suggested that these isotopic offsets would result from an isotopic fractionation caused by root morphological adaptations to xeric or saline environments that would force the water flow through the symplastic (cell-to-cell transport through walls and membranes) rather than the apoplastic (extracellular) pathway (Ellsworth and Williams, 2007; Poca et al., 2019). However, in the past decade, many studies have reported similar isotopic offsets between plant and source water in various biomes, including plants typical of temperate and humid ecosystems (Barbeta et al., 2019; Brooks et al., 2010; Brum et al., 2019; Carrière et al., 2020; De Deurwaerder et al., 2018; Evaristo et al., 2016; Geris et al., 2015; Tetzlaff et al., 2021), in addition to controlled experiments (Barbeta et al., 2020; Vargas et al., 2017). Much of this literature overlooks these plant source water isotopic offset (Anderegg et al., 2013; Muñoz-Villers et al., 2018), whereas other studies acknowledge these offsets and attribute them to either missing water sources not sampled in the field (Bowling et al., 2017) or to the isotopic separation of water pools in the soil (Brooks et al., 2010; Vargas et al., 2017). Importantly, failing to identify the cause of these plant source isotopic offsets can lead to biased estimates of plant water use from potential sources, including an overestimation of groundwater use by vegetation (Barbeta et al., 2019; Zuecco et al., 2022).
The first mechanism proposed to explain plant source isotopic offsets was that isotopic fractionation occurred during the cell-to-cell transport of water molecules through water channels (aquaporins) in the root endodermis, which would discriminate against 2H (Ellsworth and Williams, 2007; Mamonov et al., 2007; Poca et al., 2019). More recently, a series of studies has identified other plausible causes. For example, methodological artefacts associated with water extraction or isotope analysis protocols could cause apparent fractionation. It is known that the water isotopic composition of plant and soil water samples measured using laser-based instruments can be biased due to contamination of the absorption spectra by organic compounds (Brand, 2010; Schmidt et al., 2012; West et al., 2010). For this reason, spectral corrections have been developed for these laser-based instruments (Martín-Gómez et al., 2015), and reproducible results have been demonstrated for soil and plant samples measured with laser and mass spectrometers (Bowling et al., 2017; Barbeta et al., 2022). Potential issues associated with water extraction protocols are more complicated to harmonise, particularly for cryogenic vacuum distillation (CVD, Orlowski et al., 2018). Besides parameters inherent to the CVD protocol (mainly, extraction time, temperature, and vacuum line pressure), soil texture, cation exchange capacity, and organic matter content have been shown to affect the isotopic composition of extracted soil water (Chen et al., 2021; Orlowski et al., 2018). Alternatives to CVD exist for soil samples, such as water extraction with suction lysimeters (e.g Carrière et al., 2020) or online measurements of liquid-vapour equilibration (Dubbert et al., 2013), but CVD is still, by far, the most common methodology (Amin et al., 2020). The isotopic composition of stem water could also be altered following CVD, as a hydrogen exchange between water and cellulose during the extraction should cause a systematic, and potentially significant, depletion of the extracted water in 2H (Chen et al., 2020). Apparent fractionation could also be caused by within-stem isotopic heterogeneity created by isotopic surface effects in soil (G. Chen et al., 2016) and stem (Barbeta et al., 2022) water pools. In studies where sap water was extracted more directly – either by taking advantage of positive root pressure (Zhao et al., 2016), by using mechanical squeezing with the use of a Scholander pressure chamber (Geißler et al., 2019; Magh et al., 2020; Zuecco et al., 2022), or directional centrifugation along the stem main axis using a Cavitron apparatus (Barbeta et al., 2022) – no significant isotopic offsets were found between sap and source water. In addition, the CVD-extracted water remaining in non-conductive tissues and bulk stem water have both been shown to be depleted in 2H relative to sap water (Barbeta et al., 2022; Zuecco et al., 2022). These recent findings would suggest that isotopic offsets would be more likely when water contained in non-conductive tissues constituted a larger proportion of bulk stem water, for example, under water stress or in species with few small xylem vessels. Most often, detailed measurements of these anatomical traits are only available for discrete study sites (e.g. Cosme et al., 2017), but fortunately, other proxies of anatomical traits like wood density or parenchyma fraction are more widely available (Chave et al., 2009; Morris et al., 2018). In addition, isotopic enrichment of stem water above source water can result from evaporative enrichment caused by water loss through the bark under hot and dry conditions (Martín-Gómez et al., 2017). Importantly, none of these mechanisms are mutually exclusive; for example, Barbeta et al. (2020) found that the isotopic offset between plant and source water in potted saplings disappeared under water-limited conditions, and argued that this was caused by a combination of surface isotopic effects in soil and stem water pools, with evaporative enrichment of stem water as a result of the reduction in sap flow. A systematic characterization of the global patterns of these plant source isotopic offsets and their correlations with abiotic and biotic drivers would be the first step towards identifying their most likely underlying mechanisms.
Scattered evidence across the literature suggests that the mismatch in isotopic composition between plant and source water could be more widespread than previously assumed, but we still lack a systematic quantification of its extent and variability. In this study, isotopic offsets between plant and source water are quantified by means of the line conditioned excess (LC-excess, Landwehr and Coplen, 2006) and analogous metrics (Barbeta et al., 2019). A negative LC-excess indicates that the plant accesses water that has undergone evaporative enrichment, for example, shallow soil water (Zhao et al., 2020). Given that water stored in the soil is the most likely water source for the vast majority of plants (Amin et al., 2020), to detect isotopic mismatches between plant and source waters, we should compute the δ2H offset between plant water and its corresponding soil water line (SW-excess, Barbeta et al., 2019) in addition to the LC-excess. When we found that estimated values of both SW-excess and LC-excess for a given plant were significantly different from zero, this would indicate that there could be a genuine mismatch in isotopic composition between plant water and its most likely sources (e.g. Tetzlaff et al., 2021). Here, we calculated LC-excess and SW-excess values from a compilation of 112 studies reporting the dual water isotopic composition of plant (stem) and source waters, and analysed their relationship with environmental and climatic conditions (air temperature and soil moisture content). In addition, we also assessed the influence of ecologically relevant factors, including mycorrhizal habit and plant functional traits, mediating nutrient and water-use strategies (W. Chen et al., 2016; Flo et al., 2021), as well as the comparison between taxonomic groups (angiosperms vs. gymnosperms) with known distinct hydraulic architecture and functioning (Johnson et al., 2012). Our aim was to quantify potential isotopic offsets between plant and source water, and their relationship with biotic and abiotic drivers. We sought to test whether these offsets were likely driven by methodological, biological, or abiotic factors. We expected to find significant correlations between these offsets and environmental or biological drivers, which should help identify their possible underlying mechanisms. In contrast, a lack of significant correlations could suggest that methodological artefacts (mainly due to CVD) would be more likely to be the main cause of these offsets. Following previous arguments (Barbeta et al., 2019; Poca et al., 2019), we hypothesised that plant source isotopic offsets (i) would not be restricted to xeric and saline environments, and instead, would be found across all biomes, also, (ii) these offsets would increase in plants with a higher fraction of stem water in non-conductive tissues (i.e. under low water availability and in species with higher wood density and parenchyma fraction), and (iii) would be more likely in plants that are known to establish mutualistic relationships with mycorrhizal fungi.
2.1 Plant and source water isotopic composition
To compile a data set reporting the dual water isotopic composition for plant and soil samples, first, we pooled and reviewed studies included in three previous meta-analyses (Amin et al., 2020; Barbeta and Peñuelas, 2017; Evaristo and McDonnell, 2017). We then conducted a bibliographic search for peer-reviewed papers published after 2016 on Scopus, Web of Science, and Google Scholar. The search was performed in December 2020 using the terms (water AND isotop*) AND (dual OR (hydrogen AND oxygen)) AND (plant OR tree OR vegetat*). After screening the title and abstract, we selected studies that reported (1) plant (stem) and source water isotopic composition, including the soil; (2) δ18O and δ2H for both plant (stem) and source water; and (3) sufficient simultaneous soil data (n≥3) to fit a soil water line (SWL). Our final database contained 112 studies. For each study, the isotopic composition (δ18O and δ2H) of plant (stem) and source water was obtained from the associated published data sets, provided by the corresponding author/s or extracted from figures in the article using WebPlotDigitizer (Rohatgi, 2020). Plant water included water extracted from wood cores, lignified stems, and rhizomes, never leaves or other transpiring tissues, and hereafter is referred to as “stem water”. Source water was soil water and meteoric waters. For all studies, we recorded ancillary data including information of the study site, methodology, and study species. Information of the study site included the geographic location of the sampling sites (latitude, longitude, and elevation), climate (mean annual temperature and precipitation), slope and intercept of the local meteoric water line (LMWL), and study type (experimental studies on potted plants under controlled conditions, observational studies in irrigated urban gardens or agricultural fields, and observational studies under natural conditions). For those studies that did not report the slope and intercept of the LMWL, these parameters were calculated from estimates of the isotopic composition of precipitation obtained from the Online Isotopes in Precipitation Calculator (OIPC3.0, Bowen, 2017; Bowen et al., 2005; IAEA/WMO, 2015). Information on the methodology included the soil water extraction method (suction lysimeter, direct equilibration, or vacuum distillation, including CVD, azeotropic vacuum distillation, and other similar methodologies), plant water extraction method (direct xylem water extraction, direct vapour equilibration or vacuum distillation), and the instrument type used for the analyses of water isotopic composition (mass spectrometer or laser spectrometer). From the 112 studies reviewed, 94 studies used vacuum distillation (mainly CVD) to extract both stem and soil water. For extraction of soil water, 10 studies combined vacuum distillation and suction lysimeters (Dwivedi et al., 2020; Geris et al., 2015, 2017; Grossiord et al., 2017; Hervé-Fernández et al., 2016; Li et al., 2020; Marttila et al., 2018; Muñoz-Villers et al., 2018; Nehemy et al., 2020; Snelgrove et al., 2021) and four studies used only suction lysimeters (Jespersen et al., 2018; Lovelock et al., 2017; Yin et al., 2015; Zhang et al., 2011). For stem water, 108 studies used vacuum distillation, two studies used mechanical squeezing with the use of a Scholander pressure chamber (Geißler et al., 2019; Magh et al., 2020), one study used hand-pump suction (Jiménez-Rodríguez et al., 2019), and one study used direct equilibration of liquid-vapour for both soil and stem water (Bertrand et al., 2014). Vacuum distillation was the most common methodology for water extraction of soil (93 %) and stem water (96 %). Hence, our database did not allow for a robust analysis of the potential effects of water extraction methodology on plant-source isotopic offsets. Information of the study species included the species name, taxonomic group (angiosperm or gymnosperm), leaf habit (deciduous, semi-deciduous, or evergreen), leaf shape (broadleaved or narrow-leaved), and growth form (tree, shrub, or non-woody). We also recorded the sampling date of the sampling event (month and year or at least year) and plot within the study site. For our analyses, we considered that the experimental unit was the sampling “campaign”. We defined a campaign as a data collection event that occurred within a study site (or plot) within a limited time interval, thus, each study consisted of one or more campaigns depending on the number of sampling events. Our initial database consisted of 112 studies, but only 102 studies were finally used in the analysis (Table 1, see Sect. 2.2 for further details).
Table 1List of studies with the number of campaigns for which SW-excess and LC-excess were calculated with the number of plant species sampled, country where the study plots were located (G.E. indicates the glasshouse experiment), number of sampling plots, number of sampling events, and number of campaigns. Asterisks (*) next to the number of species indicate that although several species were sampled within a study, it was not possible to ascribe distinct water isotopic compositions to each species.
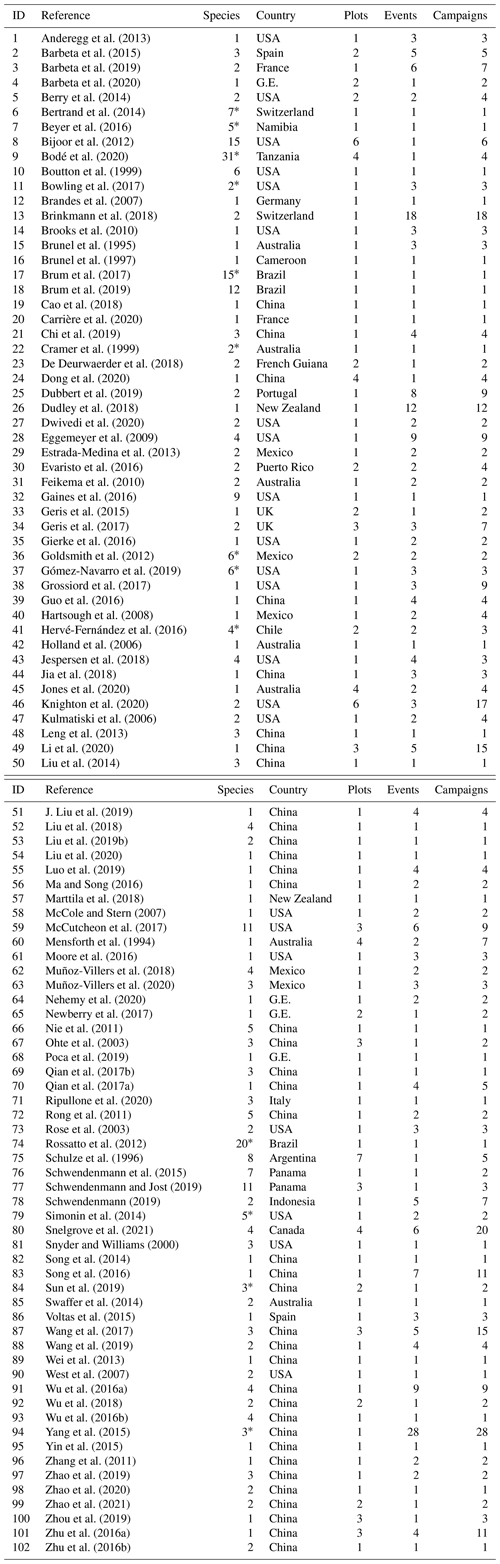
2.2 Calculation of SW-excess and LC-excess
We fitted a soil water line (SWL) for the isotopic composition of soil water from samples collected within each campaign (observations within a study, sampling event, and plot) according to Eq. (1) (Sprenger et al., 2016):
where δ2Hs and δ18Os correspond to soil water samples from various depths, locations, or pots (in the case of glasshouse experiments), within a site (or plot), sampling event, and study. Fitted parameters as and bs are the slope and intercept of the SWL. Parameters as and bs were calculated only for those campaigns where the linear relationship between δ2Hs and δ18Os was significant (P < 0.05). In this step, we discarded 133 campaigns corresponding to nine studies (Geißler et al., 2019; Huang and Zhang, 2015; Liu et al., 2011; Lovelock et al., 2017; Magh et al., 2020; McKeon et al., 2006; Saha et al., 2015; Su et al., 2020; Twining et al., 2006). Next, we estimated the difference in δ2H between each plant water sample and its corresponding soil water line (SW-excess) according to Eq. (2) (Barbeta et al., 2019):
where δ2Hp and δ18Op denote the isotopic composition of individual plant water samples. We calculated a value of SW-excess for each plant sample, and averaged values within species and campaigns. We discarded 14 campaigns because they lacked their corresponding observations for plant water. In addition, of the remaining 103 studies, all but one (Jiménez-Rodríguez et al., 2019) reported isotopic composition of bulk plant water and thus, this study was not included in the end. The final number of studies included was 102, with 407 campaigns and 197 species.
To measure how well defined the SW-excess is for a given species and campaign, we computed the standard error of the mean of SW-excess (σSW-ex), according to Eq. (3) (Taylor, 1997):
where and are the standard errors of the slope and intercept of the SWL, respectively, and σ2Hp and σ18Op are the standard errors of the mean (per species and campaign) of δ2Hp and δ18Op, respectively. To characterize how dispersed SW-excess was for a given campaign, we also calculated the variance of SW-excess according to Eq. (4):
where Var() and Cov() denote the variance and covariance of variables. For studies reporting one value of δ2Hp and δ18Op per species or campaign, we estimated their variance from the means of all other calculated σ2Hp, σ18Op, and cov2Hp, 18Op.
Similar statistics were derived for the line conditioned excess (LC-excess), according to Eq. (5) (Landwehr and Coplen, 2006):
where aL and bL are the slope and intercept of the corresponding LMWL. We calculated a value of LC-excess for each plant sample (only for observational studies), and then averaged values within species and campaigns. The standard error of the mean (σLC-ex) and variance [Var(LC-excess)] of LC-excess was calculated as in Eqs. (3) and (4), but assuming that and were zero. For each campaign, we considered that either the LC- or SW-excess was different from zero when its estimate plus or minus its standard error was greater or smaller than zero.
2.3 Climatic, environmental, and biological data
Climatic and environmental data were extracted from the ERA5-Land Copernicus data service (Hersbach et al., 2019) and downloaded from the Copernicus Climate Change Service (C3S) Climate Data Store. For each study site and sampling event, we extracted: monthly and annual air temperature at 2 m above the surface, monthly and annual total precipitation, monthly and annual potential evapotranspiration, monthly and annual soil volumetric water content (VWC) at four depth intervals (0–7, 7–28, 28–100, and 100–289 cm), and average soil water content for the upper 100 cm (calculated from soil VWC of the upper soil layers: 0–7, 7–28, and 28–100 cm). In addition, for each study site, we extracted soil class from the ERA5 database according to the FAO/UNESCO Digital Soil Map of the World (FAO and UNESCO, 2003). This database classifies the soil within each ∼10 km pixel into seven soil classes of coarse, medium, medium fine, fine, very fine, organic, and tropical organic. Finally, for each plant species, we obtained average values of wood density (Chave et al., 2009, available for 65 species, representing 258 out of the 656 observations), parenchyma volume fraction (Morris et al., 2018, available for 26 species of angiosperms, representing 150 out of the 656 observations), and mycorrhizal habit, i.e. whether a certain plant species has been reported to establish a symbiotic association with either an arbuscular or ectomycorrhizal fungi, both types of fungi, or none of them (from Maherali et al., 2016, available for 118 species, representing 408 out of the 656 observations).
2.4 Statistical analyses
Our final database consisted of 656 records of mean values of SW-excess and 642 of LC-excess (the LC-excess was not calculated for glasshouse studies) for 197 species and 407 campaigns gathered from 102 studies. We used linear mixed models (LMMs) to assess the effects of biotic and abiotic variables on the slope of the SWL, SW-excess, and LC-excess, including study as a random factor. To assess the global prevalence of isotopic offsets between plant water and its potential sources, first, we ran LMMs without fixed factors (null models). Next, in the fixed part of the model, we included the following potential explanatory variables: mean monthly air temperature annual potential evapotranspiration, monthly and annual precipitation, mean monthly soil VWC, soil class, and methodology used for analyses of water isotopic composition for the slope of the SWL, LC-excess, and SW-excess; and wood density, fraction of parenchyma, leaf habit, growth form, leaf shape, mycorrhizal habit, and taxonomic group for LC-excess and SW-excess. All explanatory variables were included in our LMMs in standardised form. In addition, for the LMMs assessing potential effects of plant traits measured at the species level (wood density, parenchyma fraction, and mycorrhizal habit), species identity was included as a random factor of the model because some species were measured in multiple studies. We performed individual models for each explanatory variable, and those that had significant effects were tested in combination in additive models. Estimated effects for the SWL slope, LC-excess, and SW-excess were weighted by the inverse of the variance to consider the precision of the information given by each study (Koricheva et al., 2013). In meta-analytical models, two potential sources of variation might be accounted for. These are the random sampling variability within each study (i.e. within-study heterogeneity) and the additional variability between studies caused, for instance, by different experimental conditions (i.e. between-study heterogeneity). Thus, we calculated a heterogeneity statistical index to test the percentage of variation across studies caused by between rather than within-study heterogeneity (I2, Higgins and Thompson, 2002). The 95 % confidence intervals of the I2 indices were 96.6 %–99.61 %, 99.85 %–99.851 %, and 99.98 %–99.981 % for the SWL slope, LC-excess and SW-excess, respectively, indicating that most variation corresponded to between-study heterogeneity. We selected the LMMs including random effects (i.e. accounting for both between and within-study heterogeneity) rather than those with only fixed effects (i.e. only accounting for within-study heterogeneity), as they fitted the data better in terms of the Akaike information criterion (AIC) (Burnham and Anderson, 2002). Therefore, both within and between-study heterogeneities were included in the models. In addition, to disentangle direct and indirect effects of environmental variables on the SW-excess, we ran additional mixed models. We aimed to assess whether any observed effect on the SW-excess was caused by a preceding effect of the same variable on the SWL parameters (slope and intercept). Indeed, those linear regression parameters are used to calculate the SW-excess and they could be potentially affected by environmental variables. Therefore, we extracted the residuals of the correlations of the SW-excess with the SWL parameters, and subsequently introduced them in a model with the relevant environmental variables. This way, only those effects that would be significant in this second model (using residuals as a response variable) could be considered as direct environmental effects on the SW-excess. All analyses were performed in R (version 4.0.3, R Core Team, 2020) using the following packages: lme4 (Bates et al., 2015), lmerTest (Kuznetsova et al., 2017), MuMIn (Barton, 2009), standardize (Eager, 2017), emmeans (Searle et al., 1980) and performance (Lüdecke et al., 2020)
3.1 Combined analysis of SW-excess and LC-excess
We compared SW-excess and LC-excess within species and campaigns (glasshouse experiments excluded, since LC-excess could not be calculated), and found that SW-excess was negative for 184 of our records (out of 642 records, with glasshouse studies excluded). We found that for 95 % of these records (175 out of 184), LC-excess was also negative (Fig. 1). In these 175 cases, there would be a mismatch in the isotopic composition between stem and source water, regardless of whether plants were taking up precipitation, groundwater, streamflow, or water stored in the soil that had been subject to evaporation enrichment. These 175 cases were distributed across 57 of the 153 study sites with no apparent bias linked to geographical region (Figs. 2 and 3). In addition, we found 12 records for which both SW-excess and LC-excess were positive.
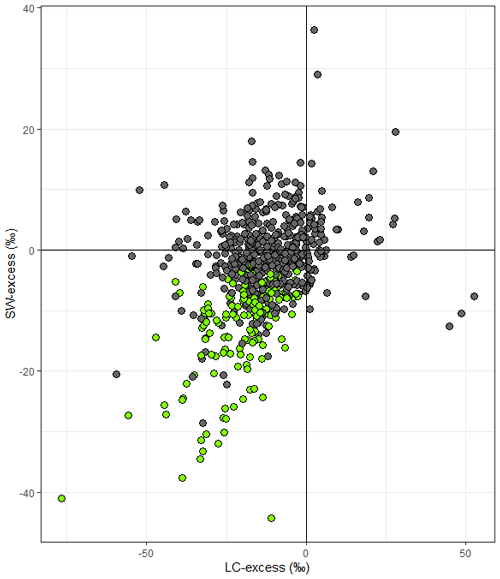
Figure 1Soil water excess (SW-excess) plotted against line conditioned excess (LC-excess). Points are the mean values per species and campaign (observations from a given sampling event and plot within a study). Green symbols indicate observations where both LC-excess and SW-excess plus their corresponding standard error were negative. Error bars have been omitted for clarity. Note that the scales of the x and y axes are different.
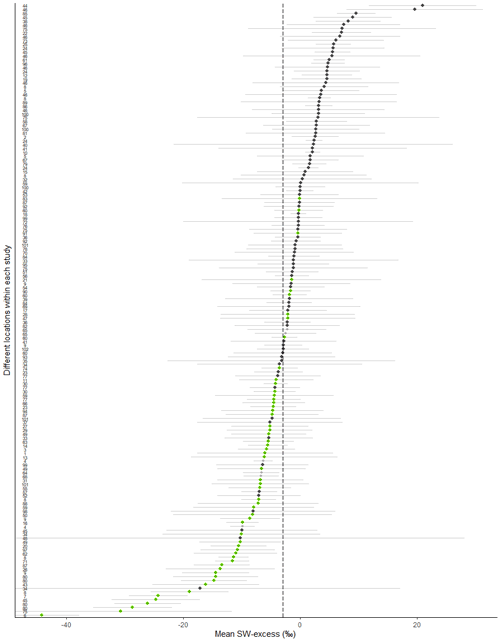
Figure 2Mean (±SE, n= number of records per study site) soil water excess (SW-excess) for each study site. The dotted line is the overall mean estimate of SW-excess (−3.02 ‰) according to the null linear mixed model. Each dot corresponds to a study site (see ID numbers on the left and in Table 1 for the corresponding references). Filled green dots depict study sites where both the line conditioned excess (LC-excess) and SW-excess (plus their corresponding SE) were negative for at least one campaign and dark grey dots depict study sites where LC-excess and/or SW-excess were not different from zero for all campaigns. Colourless dots depict glasshouse studies.
3.2 Overall value and effects of abiotic variables on the slope of the soil water line (SWL)
The linear regression between δ2H and δ18O of soil water samples was significant for 422 of the 555 campaigns compiled initially. According to the results of the null LMM (Table 2) and considering the weight and the random variability across studies, the overall mean SWL slope was significantly positive and lower than that of the global meteoric water line (P < 0.001). Of all campaigns for which the SWL regression was significant, the mean SWL slope was 5.52±0.17 (±SE), according to estimates from the null model, and the mean intercept was ‰. The results of the LMMs, including climatic and environmental variables in the fixed part of the model, indicated that the slope of the SWL was sensitive to various climatic drivers. The slope of the SWL decreased with warmer temperatures (Table 2). In contrast, the slope of the SWL increased with soil VWC of the upper soil layers (Table 2) and with integrated soil water content (Table 2). Finally, the slope of the SWL also increased with annual and monthly precipitation (Table 2). We did not find any significant differences in the SWL slope among soil classes (Table S2). The methodology for measuring soil water isotopic composition (mass vs. laser spectrometers) did not have any significant effect on the estimated SWL slope (P= 0.327 Table S2).
3.3 Overall estimates of line conditioned excess (LC-excess) and effects of biotic and abiotic variables
We calculated 642 mean values of LC-excess from 400 campaigns (campaigns from glasshouse studies excluded), 98 studies, and 194 species. The overall mean value of LC-excess was significantly negative ( ‰, P < 0.001 from the LMM with no fixed effects), indicating that, overall, plant water samples fell below their corresponding LMWL in the dual isotope space. The annual potential evapotranspiration had a positive effect on LC-excess (P < 0.001). There were some differences in LC-excess among soil classes (P= 0.028); e.g. LC-excess was less negative in organic than in medium texture soils, although our database only included five observations for the soil class “organic” (Table S2). LC-excess also differed among plant types according to mycorrhizal habit (P= 0.014); e.g. values of LC-excess were more negative in plants that have been shown to form associations with both types of mycorrhizal fungi (Table S2), while no differences were found between ectomycorrhizal or arbuscular associations (P= 0.999). We did not find significant differences between LC-excess values calculated from measurements of stem water isotopic composition measured with mass- or laser spectrometers (P= 0.421).
3.4 Overall estimates of soil water excess (SW-excess) and effects of biotic and abiotic variables
We calculated 656 mean values of SW-excess from 407 campaigns and 197 species, using observations of stem water isotopic composition and the slope and intercept of their corresponding SWL. The overall mean estimate of SW-excess was significantly negative ( ‰, P < 0.001 according to the LMM with no fixed effects), indicating that there was an overall significant isotopic offset between stem and soil water.
We found that there was a significantly positive relationship between SW-excess and monthly air temperature (P < 0.001; Fig. 4a) and monthly potential evapotranspiration (PET; P= 0.002; Supplement Fig. S1a), and a significantly negative relationship between SW-excess and mean monthly soil VWC of the upper soil layers (0–7, 7–28, and 28–100 cm; P < 0.001; Fig. S1b–d), but not with soil VWC from deeper soil layers (Table S1). SW-excess was also significantly and negatively correlated with integrated soil water content for the upper (0–100 cm) soil (P < 0.001; Fig. 4b). Neither monthly nor annual precipitation was significantly correlated with SW-excess (Table S1). When assessed in combination, we found that monthly air temperature still had a significantly positive correlation with SW-excess (P < 0.001), but soil water content did not (P= 0.083). Importantly, a more detailed analysis of the residuals of the relationship between SW-excess and the SWL parameters (slope and intercept) revealed that only the temperature effects had a direct effect on SW-excess (Table S3). On the other hand, the observed effects of soil VWC on SW-excess appeared to be a consequence of the direct effect of soil VWC on the SWL slope and intercept (Table S3).
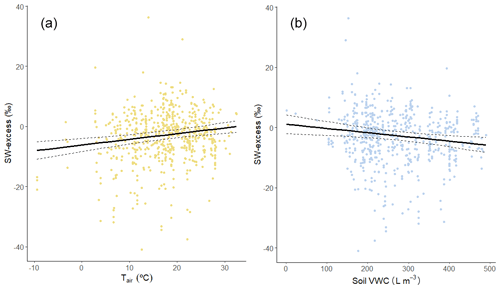
Figure 4Soil water excess (SW-excess) plotted against (a) mean monthly air temperature (Tair) and (b) monthly soil volumetric water content (VWC) of the upper 100 cm. Each point is the mean SW-excess per species and campaign (observations from a given sampling event and plot/site within a study). The solid and dashed lines are the prediction and standard error of the corresponding linear mixed model, with a single predictor variable.
Table 2Results (t and P), sample size (n), and estimated slope (except for the null model, where “estimate” is the intercept) and standard error, according to the linear mixed models (including the null models without any predictor variables) to assess the effects of temperature, soil volumetric water content (VWC), and potential evapotranspiration (PET) on the slope of the soil water line (SWL slope), line-conditioned excess (LC-excess), and soil water excess (SW-excess). All parameter estimates have been standardised. Only models with significant results are shown.
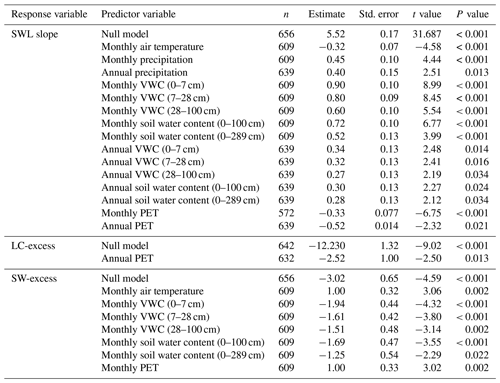
According to our results, the mean SW-excess did not differ among soil classes (Table S2). We did not find any significant difference between plant groups (Table S2); mean values of SW-excess did not differ between angiosperms and gymnosperms (P= 0.73), nor among growth forms (trees, shrubs, and non-woody plants, P= 0.07), leaf habit (deciduous, evergreen, or semi-deciduous, P= 0.63) or leaf shape (broad or narrow, P= 0.51). Also, we did not find significant differences among plant groups according to their presumed mycorrhizal habit (P= 0.64). For those species for which we had estimates of wood density and/or parenchyma fraction, the LMMs (including species identity in the random part of the model) did not reveal any significant relationship of any of these wood anatomical variables with SW-excess (Table S1). Mean (±SE) SW-excess values estimated from studies using either mass or laser spectrometers to measure stem water isotopic composition were both significantly negative at ‰ and ‰ for mass and laser spectrometers, respectively, but there was a significant difference between instrument types (P= 0.048). Similarly, the type of instrument used to measure soil water isotopic composition also had a significant effect on SW-excess (P= 0.015, and for mass and laser spectrometers, respectively). Finally, for those studies that included mobile soil water (soil water samples obtained with suction lysimeters or similar), the estimated SW-excess was not significantly different from that of studies extracting all soil water with cryogenic vacuum distillation (P= 0.64).
Our meta-analysis revealed that the isotopic composition of plant (stem) water did not always faithfully reflect its most likely source, and this was evident from results from many different types of biomes. The deviation in isotopic composition of stem water from soil water varied substantially in size and direction, but, on average, it was slightly more negative than soil water. The isotopic depletion of plant water relative to its source was originally thought to be restricted to arid or saline environments (e.g. Ellsworth and Williams, 2007; Lin and Sternberg, 1993). However, we show here that sites depicting significantly negative SW-excess (i.e. where plant water is isotopically depleted with respect to its most likely source, i.e. the soil) are widespread, and span temperate, boreal, and tropical ecosystems. The combined analysis of SW-excess and LC-excess showed that for the majority (95 %) of cases where SW-excess was negative, LC-excess was also negative. This result indicates that plant water uptake from sources other than soil water that have not undergone evaporative enrichment (such as groundwater) cannot explain the observed mismatch in isotopic composition between plant and soil water. Instead, our results agree with those of recent studies (Barbeta et al., 2022; Chen et al., 2020) that call into question the general assumption that bulk plant water faithfully reflects the isotopic composition of its source.
We compiled 112 studies reporting the dual isotopic composition of plant water and its sources, and we estimated values of SW-excess for 102 of them and values of SW-excess and LC-excess for 98 of them. These estimates were widely distributed across the globe, encompassing boreal, tropical, temperate, Mediterranean, arid, and semi-arid ecosystems. However, overall, there is a literature bias towards data collection in temperate forests, consequently, these ecosystems were overrepresented in our database. In contrast, observations from tropical ecosystems were the scarcest, in line with observations from previous meta-analyses of stable isotope data of plant water and its sources (Amin et al., 2020; Barbeta and Peñuelas, 2017; Evaristo and McDonnell, 2017). Here, we aimed to partially overcome the limited climatic variability represented by biome type and geographic location by incorporating seasonal climatic variability, specific to each study site, when available. To do so, we gathered monthly values of air temperature and soil water availability for each study plot and sampling campaign, encompassing a large breadth of climatic values spanning from −10 up to 35 ∘C for mean monthly air temperature and from 1 % to 50 % for soil VWC. Our analyses from this data compilation agreed with predictions from classic theory. For example, we found that, globally, the slope of the soil water line (SWL) decreases as temperature increases and water availability decreases because of increased evaporative enrichment (Craig and Gordon, 1965; Sprenger et al., 2016)
At the global scale, we found positive effects of monthly air temperature and negative effects of soil VWC on the SW-excess. One of the main results from our analysis was that the SW-excess was clearly most negative in cooler and wetter environments. This result is in agreement with recent observations from an array of boreal forests, where significant offsets (i.e. negative SW-excess) were found in all study sites, with the two coldest sites depicting the most negative values of SW-excess (Tetzlaff et al., 2021). The SW-excess is calculated with the slope and intercept of the soil water line, but, in turn, those parameters correlate with soil VWC and air temperature (see above). Therefore, the subsequent analysis of the residuals that teased apart direct and indirect environmental effects on SW-excess (Table S3) revealed that the negative effect of soil VWC on the SW-excess was mediated by the variability in the parameters of the SWL. In moist sites, the slope of the SWL was steeper and closer to that of the LMWL, and the intercept took relatively more positive values, in agreement with findings like those of Benettin et al. (2018), which resulted in more negative estimates of SW-excess. On the other hand, air temperature appeared to affect SW-excess more directly (Fig. 4a). Similarly, in cold sites, the effect of low soil water evaporative enrichment could have resulted in steeper SWL slopes and hence, more negative SW-excess (see Eq. 2). Meanwhile, on the opposite end of the temperature range, warm temperatures could be causing greater evaporative enrichment of stem water (Martín-Gómez et al., 2017) and hence, partially or completely compensate the negative values of SW-excess (Barbeta et al., 2019). Overall, our results suggest that, on their own, stem evaporative enrichment (as in Martín-Gómez et al., 2017) could not explain these observed isotopic offsets because these were largest in cold and wet places. Instead, other processes sensitive to temperature and soil moisture would be causing these offsets in the field. Recent studies have shown that stem water not participating directly to the transpiration stream is more depleted than sap water flowing through xylem vessels (Barbeta et al., 2022). Although stem water content was generally not available for the studies reviewed, it would be reasonable to expect that total stem water content would have been higher in cool and moist environments (Li et al., 2021). Contrary to our expectations, these results could suggest that larger values of total stem water content could have resulted into a greater δ2H-depletion of bulk stem water. In addition, the use of internal water storage can lead to lags between root water uptake and transpiration (Knighton et al., 2020), which could result into an apparent separation of source and stem water in the dual-isotopic space. The effect of stem water content on plant source isotopic offsets could not be tested in this study, but it is noteworthy that cool and/or wet environments tend to store larger amounts of water in woody tissues (Li et al., 2021).
Isotopic mismatches between sources and plant water have been identified in glasshouse and field experiments (e.g. Tetzlaff et al., 2021; Barbeta et al., 2020; Chen et al., 2020; Vargas et al., 2017). Previously, these have been attributed to fractionation processes occurring along the soil-plant-atmosphere continuum, mostly related to hydrogen isotopes. Our meta-analysis confirms this pattern at the global scale, but cannot pinpoint a definitive mechanistic explanation. A recent study suggested that methodological artefacts related to the 2H exchange with cellulose during cryogenic vacuum extraction could be at the origin of these negative SW-excesses (Chen et al., 2020), at least in studies where plant stem water was extracted using CVD. Following the latter mechanistic explanation, the relative depletion in 2H of stem water should be associated with stem water content; plants with a lower stem water content should show more negative SW-excess values (Chen et al., 2020). If this was the case, one would expect that plants growing in drier sites with presumably lower stem water content should depict a more negative SW-excess. Contrary to our expectations, our results did not support this hypothesis and, in fact, we found that values of SW-excess tended to be less negative (smaller) in drier sites. Measurements of stem relative water content (RWC) are often collected during CVD to assess extraction efficiency (e.g. West et al., 2006), but these are rarely reported. In the future, it would be desirable that studies presenting the water isotopic composition of different plant organs also reported their RWC in a routinely manner, even more so given the increasing recognition of the functional relevance of this plant trait (Martinez-Vilalta et al., 2019; Sapes and Sala, 2021). In the database compiled here, cryogenic vacuum distillation (CVD) was the most common methodology used for extraction of both stem and soil water, as in Amin et al. (2020). Additional recent studies under controlled conditions also suggest that during CVD, isotopic exchange both within the soil and the stem could cause apparent isotopic fractionation (Adams et al., 2020; Chen et al., 2021; Orlowski et al., 2018). However, our meta-analysis did not allow us to test for the effects of extraction methodology on plant source isotopic offsets due to the paucity of studies applying alternative methodologies to CVD, since alternative methodologies based on centrifugation (Barbeta et al., 2022) or low-suction (Geißler et al., 2019; Magh et al., 2020; Zuecco et al., 2022) have only emerged recently. To help identify apparent fractionation caused by artefacts associated with CVD, future studies applying these novel methodologies should consider combining them with analyses of cryogenically extracted water from a concurrent subset of their samples (Geißler et al., 2019; Marshall et al., 2020). In addition, isotopic offsets between plant water and its sources are often attributed to soil properties underlying methodological artefacts, particularly also during CVD. Soil properties that can affect water isotopic composition measured following CVD include organic matter, texture, and cationic exchange capacity (Adams et al., 2020; Araguás-Araguás et al., 1995; Chen et al., 2021). In our meta-analysis, we compiled all the soil properties provided in the studies revised, but there were large inconsistencies across studies in the type of data used to describe soil properties. Hence, we opted to use soil classes derived from the Digital Soil Map of the World (FAO and UNESCO, 2003), downloaded from the ERA5 service (Hersbach et al., 2019). Here, we did not find significant differences among soil classes in either the slope of the SWL, LC-, or SW-excess. However, we acknowledge that the soil classes used here may not be representative of the actual soil properties at each study site due to their coarse spatial resolution (∼10 km). In the future, it would be desirable that studies analysing soil water isotopic composition systematically reported at least the following soil properties, such as soil texture (preferably by providing percentages of sand, silt, and clay), cationic exchange capacity, and organic matter or total carbon, instead of merely stating the soil type or texture. Finally, our study does not completely discard potential biases in water isotopic composition associated with the type of instrument used for measuring water isotopic composition (mass vs. laser spectrometers). However, instrument type cannot explain the negative overall estimate of SW-excess, as estimates from either type of instrument were significantly negative. Our results showed that plant source water isotopic offsets depict significant relationships with climatic drivers, and suggest that methodological artefacts associated with isotopic measurements and cryogenic vacuum extractions are highly unlikely to be the sole mechanisms explaining the observed source-stem water isotopic offset.
The combined analyses of LC-excess and SW-excess can help identify the type of ecosystems where we could expect larger biases on the attribution of plant water sources from water isotopic composition. For example, in cold and/or very wet climates where soil water is subject to very little evaporative enrichment, the slopes of LMWL and SWL are similar, and neither LC-excess nor SW-excess were different from zero, then variations in plant water isotopic composition would likely track that of its most likely source, i.e. precipitation water (e.g. Geris et al., 2015). In arid or semi-arid ecosystems where deep-rooted vegetation has access to groundwater and when SW-excess is different from zero, but LC-excess is not, then we would infer that the vegetation was taking up groundwater that had not undergone evaporative enrichment (e.g. Miller et al., 2010). Conversely, in temperate ecosystems when SW-excess was not significantly different from zero and LC-excess was negative, most likely, a combined analyses of water isotopic composition of plant and soil water would reveal that plants were taking up water from the upper soil layers, where water would be subject to evaporative enrichment (Brinkmann et al., 2018). However, in hot climates, evaporative enrichment could affect plant water isotopic composition too, irrespective of the water source and of potential isotopic offsets (Martín-Gómez et al., 2017), and partially or completely compensate for potential isotopic offsets. In any case, the various possible mechanisms underlying isotopic mismatches are not mutually exclusive and multiple mechanisms exerting opposing effects could coexist, but these can only be disentangled in experiments under controlled conditions (Barbeta et al., 2020; Chen et al., 2020; Vargas et al., 2017). Still, in field studies, potential errors in the attribution of plant water sources could be avoided, or at least identified, by including analyses of both LC-excess and SW-excess. Yet, here, we emphasise that null values for either SW-excess and/or LC-excess might not necessarily imply an absence of offsets in isotopic composition between plant water and its sources, since these offsets can be masked by mechanisms with opposite effects acting simultaneously (Barbeta et al., 2020).
We expected differences among plant groups in plant source isotopic offsets based on their anatomical traits and mycorrhizal partnership. For example, water in non-conducting tissues has been shown to be depleted in 2H with respect to sap water (Zhao et al., 2016; Barbeta et al., 2022) and, thus, a greater fraction of water stored in non-conductive stem compartments could cause larger isotopic offsets between plant and source water. Our analyses did not reveal any significant difference in either LC-excess or SW-excess among plant groups according to their evolutionary history and hydraulic strategy (angiosperms vs. gymnosperms), growth form (trees, shrubs, or non-woody), leaf habit, or morphology. Our data set, however, did not encompass a balanced representation of all plant groups, for example, nearly three quarters (141 out of 197) of the species included in our meta-analysis were trees, whereas less than 20 % of our observations corresponded to non-woody species (36 out of 197). There were some differences among plant groups according to the presumed mycorrhizal partnership on LC-excess. Arbuscular mycorrhizal associations have been hypothesised to cause isotopic fractionation during root water uptake (Poca et al., 2019) and, therefore, we expected larger isotopic offsets in plants forming associations with arbuscular mycorrhizae, but our results did not support this hypothesis. We found that LC-excess, but not SW-excess, was more negative for plants that have been shown to form mycorrhizal associations with either arbuscular or ectomycorrhizal fungi. Mycorrhizal associations are beneficial for the host plant because they increase nutrient and water availability for the plant and in return, the host plant supplies carbohydrates to their mycorrhizal partner (Antunes and Koyama, 2017). Given the carbon costs of these associations for the plant, to maximise their investment return, we would expect that plants forming mycorrhizal associations would allocate larger proportions of their root and fungal hyphal biomass to the shallower soil layers where nutrient concentrations are higher (Esteban and Robert, 2001). This could explain the more negative LC-excess observed in plants forming mycorrhizal associations, as their main water source would be shallow soil water (subject to evaporative enrichment), instead of deep mobile water pools.
We also explored correlations between plant source isotopic offsets and two wood anatomical traits of wood density and parenchyma fraction for angiosperms (Morris et al., 2018). If isotopic heterogeneities within stem water pools underlie isotopic offsets (e.g. Barbeta et al., 2022; Zhao et al., 2016), then we should observe larger isotopic offsets in species where sap water constitutes a smaller fraction of total stem water, i.e. species with narrower conduits, higher parenchyma fraction, and denser wood. Our results did not agree with this prediction and suggest that anatomical traits might not be good predictors of plant source isotopic offsets. Nonetheless, our results do not discard isotopic heterogeneity within the stem as a plausible mechanism driving observed offsets. Isotopic heterogeneity between water pools within the stem can still result in isotopic offsets between bulk stem water and source water, but the extent of this offset would be determined by the actual plant relative water content at the time of measurement (Barbeta et al., 2020; Chen et al., 2020) more than the wood anatomical traits alone.
We calculated LC-excess and SW-excess from more than a hundred studies distributed globally and found that, overall, the isotopic composition of plant water did not always match its most likely sources. This isotopic offset was largest in cold and wet sites where plant water plotted below and/or to the right of source water in the dual isotope space, whereas plant water generally plotted closer to the soil water line in hot climates. Our results call into question the long-standing assumption that plant water isotopic composition faithfully reflects that of its source. Based on the recent literature, this does not seem to be the case for δ2H, at least. The significant correlations found between the magnitude of these plant source isotopic offsets with temperature and with soil moisture suggest that these offsets are unlikely caused by purely methodological artefacts, despite the variety of sampling methodologies, study sites, and range of soil sampling profiles. However, the ultimate mechanisms driving these isotopic offsets and their ecological significance can only be unveiled with experiments under controlled conditions. The results from our meta-analysis suggest that these experiments should include comparisons of contrasting soil properties, plant species with varying wood traits, and encompass gradients of plant relative water content and storage. These experiments would shed light on the most plausible mechanisms underlying these isotopic offsets and contribute to avoiding erroneous attributions of source water from analyses of water isotopic composition.
The code used for all statistical analyses is available upon request.
The data set generated and used in this publication can be accessed at https://doi.org/10.6084/m9.figshare.20079434.v1 (de la Casa et al., 2022)
The supplement related to this article is available online at: https://doi.org/10.5194/hess-26-4125-2022-supplement.
AB, TEG, LW, and JO conceived the idea and designed the study. JdlC performed the literature review and collected the data. JdlC and ARU performed the statistical analyses. JdlC and TEG wrote the first manuscript draft. All authors contributed to the writing.
The contact author has declared that none of the authors has any competing interests.
Publisher’s note: Copernicus Publications remains neutral with regard to jurisdictional claims in published maps and institutional affiliations.
We thank all the authors that kindly shared their published data and responded to our queries. Thanks to Javier Porras for his contribution to data collection and to Dominic Roye for his assistance during compilation of climatic data.
Funding was provided by the Regional Government of the Basque Country (Programa Investigación Básica y Aplicada, grant no. PIBA-2019-105) and by the Spanish Ministry of Science (grant no. PHLISCO PID2019-107817RB-I00). Additional financial support was provided by the Severo Ochoa (grant no. CEX-2018-000828) and María de Maeztu (grant no. MDM-2017-0714) excellence accreditations awarded to CREAF and BC3, respectively, by the Spanish Ministry of Science.
This paper was edited by Markus Weiler and reviewed by three anonymous referees.
Adams, R. E., Hyodo, A., SantaMaria, T., Wright, C. L., Boutton, T. W., and West, J. B.: Bound and mobile soil water isotope ratios are affected by soil texture and mineralogy, whereas extraction method influences their measurement, Hydrol. Process., 34, 991–1003, https://doi.org/10.1002/hyp.13633, 2020.
Amin, A., Zuecco, G., Geris, J., Schwendenmann, L., McDonnell, J. J., Borga, M., and Penna, D.: Depth distribution of soil water sourced by plants at the global scale: A new direct inference approach, Ecohydrology, 13, e2177, https://doi.org/10.1002/eco.2177, 2020.
Anderegg, L. D. L., Anderegg, W. R. L., Abatzoglou, J., Hausladen, A. M., and Berry, J. A.: Drought characteristics' role in widespread aspen forest mortality across Colorado, USA, Global Change Biol., 19, 1526–1537, https://doi.org/10.1111/gcb.12146, 2013.
Antunes, P. M. and Koyama, A.: Mycorrhizas as Nutrient and Energy Pumps of Soil Food Webs: Multitrophic Interactions and Feedbacks, edited by: Johnson, N., Gehring, C., and Jansa, J., Elsevier Inc., https://doi.org/10.1016/B978-0-12-804312-7.00009-7, 2017.
Araguás-Araguás, L., Rozanski, K., Gonfiantini, R., and Louvat, D.: Isotope effects accompanying vacuum extraction of soil water for stable isotope analyses, J. Hydrol., 168, 159–171, https://doi.org/10.1016/0022-1694(94)02636-P, 1995.
Barbeta, A. and Peñuelas, J.: Relative contribution of groundwater to plant transpiration estimated with stable isotopes, Sci. Rep.-UK, 7, 1–10, https://doi.org/10.1038/s41598-017-09643-x, 2017.
Barbeta, A., Mejía-Chang, M., Ogaya, R., Voltas, J., Dawson, T. E., and Peñuelas, J.: The combined effects of a long-term experimental drought and an extreme drought on the use of plant-water sources in a Mediterranean forest, Global Change Biol., 21, 1213–1225, https://doi.org/10.1111/gcb.12785, 2015.
Barbeta, A., Jones, S. P., Clavé, L., Wingate, L., Gimeno, T. E., Fréjaville, B., Wohl, S., and Ogée, J.: Unexplained hydrogen isotope offsets complicate the identification and quantification of tree water sources in a riparian forest, Hydrol. Earth Syst. Sci., 23, 2129–2146, https://doi.org/10.5194/hess-23-2129-2019, 2019.
Barbeta, A., Gimeno, T. E., Clavé, L., Fréjaville, B., Jones, S. P., Delvigne, C., Wingate, L., and Ogée, J.: An explanation for the isotopic offset between soil and stem water in a temperate tree species, New Phytol., 227, 766–779, https://doi.org/10.1111/nph.16564, 2020.
Barbeta, A., Burlett, R., Martin-Gómez, P., Fréjaville, B., Devert, N., Wingate, L., Domec, J.-C., and Ogée, J.: Evidence for distinct isotopic composition of sap and tissue water in tree stems: consequences for plant water source identification, New Phytol., 223, 1121–1132, https://doi.org/10.1101/2020.06.18.160002, 2022.
Barton, K.: Mu-MIn: Multi-model inference. R Package Version 0.12.2/r18, http://r-forge.r-project.org/projects/mumin/ (last access: 20 October 2020), 2009.
Bates, D., Mächler, M., Bolker, B. M., and Walker, S. C.: Fitting linear mixed-effects models using lme4, J. Stat. Softw., 67, 1–48, https://doi.org/10.18637/jss.v067.i01, 2015.
Benettin, P., Volkmann, T. H. M., von Freyberg, J., Frentress, J., Penna, D., Dawson, T. E., and Kirchner, J. W.: Effects of climatic seasonality on the isotopic composition of evaporating soil waters, Hydrol. Earth Syst. Sci., 22, 2881–2890, https://doi.org/10.5194/hess-22-2881-2018, 2018.
Berry, Z. C., Hughes, N. M., and Smith, W. K.: Cloud immersion: An important water source for spruce and fir saplings in the southern Appalachian Mountains, Oecologia, 174, 319–326, https://doi.org/10.1007/s00442-013-2770-0, 2014.
Bertrand, G., Masini, J., Goldscheider, N., Meeks, J., Lavastre, V., Celle-Jeanton, H., Gobat, J. M., and Hunkeler, D.: Determination of spatiotemporal variability of tree water uptake using stable isotopes (δ18O, δ2H) in an alluvial system supplied by a high-altitude watershed, Pfyn forest, Switzerland, Ecohydrology, 7, 319–333, https://doi.org/10.1002/eco.1347, 2014.
Beyer, M., Koeniger, P., Gaj, M., Hamutoko, J. T., Wanke, H., and Himmelsbach, T.: A deuterium-based labeling technique for the investigation of rooting depths, water uptake dynamics and unsaturated zone water transport in semiarid environments, J. Hydrol., 533, 627–643, https://doi.org/10.1016/j.jhydrol.2015.12.037, 2016.
Bijoor, N. S., McCarthy, H. R., Zhang, D., and Pataki, D. E.: Water sources of urban trees in the Los Angeles metropolitan area, Urban Ecosyst., 15, 195–214, https://doi.org/10.1007/s11252-011-0196-1, 2012.
Bodé, S., De Wispelaere, L., Hemp, A., Verschuren, D., and Boeckx, P.: Water-isotope ecohydrology of Mount Kilimanjaro, Ecohydrology, 13, e2171, https://doi.org/10.1002/eco.2171, 2020.
Boutton, T. W., Archer, S. R., and Midwood, A. J.: Stable isotopes in ecosystem science: Structure, function and dynamics of a subtropical savanna, Rapid Commun. Mass Spectrom., 13, 1263–1277, https://doi.org/10.1002/(SICI)1097-0231(19990715)13:13<1263::AID-RCM653>3.0.CO;2-J, 1999.
Bowen, G. J.: The Online Isotopes in Precipitation Calculator, version 3.1, https://wateriso.utah.edu/waterisotopes/pages/data_access/oipc.html (last access: 20 October 2020), 2017.
Bowen, G. J., Wassenaar, L. I., and Hobson, K. A.: Global application of stable hydrogen and oxygen isotopes to wildlife forensics, Oecologia, 143, 337–348, https://doi.org/10.1007/s00442-004-1813-y, 2005.
Bowling, D. R., Schulze, E. S., and Hall, S. J.: Revisiting streamside trees that do not use stream water: can the two water worlds hypothesis and snowpack isotopic effects explain a missing water source?, Ecohydrology, 10, e1771, https://doi.org/10.1002/eco.1771, 2017.
Brand, W. A.: Comments on “Discrepancies between isotope ratio infrared spectroscopy and isotope ratio mass spectrometry for the stable isotope analysis of plant and soil waters.”, Rapid Commun. Mass Sp., 24, 2687–2688, https://doi.org/10.1002/rcm.4685, 2010.
Brandes, E., Wenninger, J., Koeniger, P., Schindler, D., Rennenberg, H., Leibundgut, C., Mayer, H., and Gessler, A.: Assessing environmental and physiological controls over water relations in a Scots pine (Pinus sylvestris L.) stand through analyses of stable isotope composition of water and organic matter, Plant Cell Environ., 30, 113–127, https://doi.org/10.1111/j.1365-3040.2006.01609.x, 2007.
Brinkmann, N., Seeger, S., Weiler, M., Buchmann, N., Eugster, W., and Kahmen, A.: Employing stable isotopes to determine the residence times of soil water and the temporal origin of water taken up by Fagus sylvatica and Picea abies in a temperate forest, New Phytol., 219, 1300–1313, https://doi.org/10.1111/nph.15255, 2018.
Brooks, J., Barnard, H. R., Coulombe, R., and McDonnell, J. J.: Ecohydrologic separation of water between trees and streams in a Mediterranean climate, Nat. Geosci., 3, 100–104, https://doi.org/10.1038/ngeo722, 2010.
Brum, M., Teodoro, G. S., Abrahão, A., and Oliveira, R. S.: Coordination of rooting depth and leaf hydraulic traits defines drought-related strategies in the campos rupestres, a tropical montane biodiversity hotspot, Plant Soil, 420, 467–480, https://doi.org/10.1007/s11104-017-3330-x, 2017.
Brum, M., Vadeboncoeur, M. A., Ivanov, V., Asbjornsen, H., Saleska, S., Alves, L. F., Penha, D., Dias, J. D., Aragão, L. E. O. C., Barros, F., Bittencourt, P., Pereira, L., and Oliveira, R. S.: Hydrological niche segregation defines forest structure and drought tolerance strategies in a seasonal Amazon forest, J. Ecol., 107, 318–333, https://doi.org/10.1111/1365-2745.13022, 2019.
Brunel, J. P., Walker, G. R., and Kennett-Smith, A. K.: Field validation of isotopic procedures for determining sources of water used by plants in a semi-arid environment, J. Hydrol., 167, 351–368, https://doi.org/10.1016/0022-1694(94)02575-V, 1995.
Brunel, J. P., Walker, G. R., Dighton, J. C., and Monteny, B.: Use of stable isotopes of water to determine the origin of water used by the vegetation and to partition evapotranspiration. A case study from HAPEX-Sahel, J. Hydrol., 188–189, 466–481, https://doi.org/10.1016/S0022-1694(96)03188-5, 1997.
Burgess, S. S. O. and Dawson, T. E.: The contribution of fog to the water relations of Sequoia sempervirens (D. Don): Foliar uptake and prevention of dehydration, Plant, Cell Environ., 27, 1023–1034, https://doi.org/10.1111/j.1365-3040.2004.01207.x, 2004.
Burnham, K. P. and Anderson, D. R.: Model Selection and Multimodel Inference: A Practical Information, edited by: Burnham, K. P. and Anderson, D. R., Springer-Verlag, ISBN 978-0-387-22456-5, 2002.
Cao, X., Yang, P., Engel, B. A., and Li, P.: The effects of rainfall and irrigation on cherry root water uptake under drip irrigation, Agr. Water Manage., 197, 9–18, https://doi.org/10.1016/j.agwat.2017.10.021, 2018.
Carrière, S. D., Martin-StPaul, N. K., Cakpo, C. B., Patris, N., Gillon, M., Chalikakis, K., Doussan, C., Olioso, A., Babic, M., Jouineau, A., Simioni, G., and Davi, H.: Tree xylem water isotope analysis by Isotope Ratio Mass Spectrometry and laser spectrometry: A dataset to explore tree response to drought, Data Br., 29, 134332, https://doi.org/10.1016/j.dib.2020.105349, 2020.
Chave, J., Coomes, D., Jansen, S., Lewis, S. L., Swenson, N. G., and Zanne, A. E.: Towards a worldwide wood economics spectrum, Ecol. Lett., 12, 351–366, https://doi.org/10.1111/j.1461-0248.2009.01285.x, 2009.
Chen, G., Auerswald, K., and Schnyder, H.: 2H and 18O depletion of water close to organic surfaces, Biogeosciences, 13, 3175–3186, https://doi.org/10.5194/bg-13-3175-2016, 2016.
Chen, G., Li, X., Qin, W., Lei, N., Sun, L. Z., Cao, L., and Tang, X.: Isotopic fractionation induced by a surface effect influences the estimation of the hydrological process of topsoil, Hydrol. Process., 35, e14019, https://doi.org/10.1002/hyp.14019, 2021.
Chen, W., Koide, R. T., Adams, T. S., DeForest, J. L., Cheng, L., and Eissenstat, D. M.: Root morphology and mycorrhizal symbioses together shape nutrient foraging strategies of temperate trees, P. Natl. Acad. Sci. USA, 113, 8741–8746, https://doi.org/10.1073/pnas.1601006113, 2016.
Chen, Y., Helliker, B. R., Tang, X., Li, F., Zhou, Y., and Song, X.: Stem water cryogenic extraction biases estimation in deuterium isotope composition of plant source water, P. Natl. Acad. Sci. USA, 117, 33345–33350, https://doi.org/10.1073/pnas.2014422117, 2020.
Chi, Y., Zhou, L., Yang, Q., Li, S., and Zheng, S.: Increased snowfall weakens complementarity of summer water use by different plant functional groups, Ecol. Evol., 9, 4264–4274, https://doi.org/10.1002/ece3.5058, 2019.
Cosme, L. H. M., Schietti, J., Costa, F. R. C., and Oliveira, R. S.: The importance of hydraulic architecture to the distribution patterns of trees in a central Amazonian forest, New Phytol., 215, 113–125, https://doi.org/10.1111/nph.14508, 2017.
Craig, H. and Gordon, I.: Stable Isotopes in Oceanographic Studies and Paleotemperatures, edited by: Tongiorgi, E., Conference Publication, Laboratory of Nuclear Geology, Pisa, 1965.
Cramer, V. A., Thorburn, P. J., and Fraser, G. W.: Transpiration and groundwater uptake from farm forest plots of Casuarina glauca and Eucalyptus camaldulensis in saline areas of southeast Queensland, Australia, Agr. Water Manage., 39, 187–204, https://doi.org/10.1016/S0378-3774(98)00078-X, 1999.
De Deurwaerder, H., Hervé-Fernández, P., Stahl, C., Burban, B., Petronelli, P., Hoffman, B., Bonal, D., Boeckx, P., and Verbeeck, H.: Liana and tree below-ground water competition-evidence for water resource partitioning during the dry season, Tree Physiol., 38, 1071–1083, https://doi.org/10.1093/treephys/tpy002, 2018.
de la Casa, J., Barbeta, A., Rodríguez-Uña, A., Wingate, L., Ogee, J., and Gimeno, T. E.: Global dataset of stable isotopes in plant water and its sources, figshare [data set], https://doi.org/10.6084/m9.figshare.20079434.v1, 2022.
Dong, Z., Li, S., Zhao, Y., Lei, J., Wang, Y., and Li, C.: Stable oxygen-hydrogen isotopes reveal water use strategies of Tamarix taklamakanensis in the Taklimakan Desert, China, J. Arid Land, 12, 115–129, https://doi.org/10.1007/s40333-020-0051-4, 2020.
Dubbert, M. and Werner, C.: Water fluxes mediated by vegetation: emerging isotopic insights at the soil and atmosphere interfaces, New Phytol., 221, 1754–1763, https://doi.org/10.1111/nph.15547, 2019.
Dubbert, M., Cuntz, M., Piayda, A., Maguás, C., and Werner, C.: Partitioning evapotranspiration – Testing the Craig and Gordon model with field measurements of oxygen isotope ratios of evaporative fluxes, J. Hydrol., 496, 142–153, https://doi.org/10.1016/j.jhydrol.2013.05.033, 2013.
Dudley, B. D., Marttila, H., Graham, S. L., Evison, R., and Srinivasan, M. S.: Water sources for woody shrubs on hillslopes: An investigation using isotopic and sapflow methods, Ecohydrology, 11, e1926, https://doi.org/10.1002/eco.1926, 2018.
Dwivedi, R., Eastoe, C., Knowles, J. F., Wright, W. E., Hamann, L., Minor, R., Mitra, B., Meixner, T., McIntosh, J., Ty Ferre, P. A., Castro, C., Niu, G. Y., Barron-Gafford, G. A., Abramson, N., Papuga, S. A., Stanley, M., Hu, J., and Chorover, J.: Vegetation source water identification using isotopic and hydrometric observations from a subhumid mountain catchment, Ecohydrology, 13, 1–17, https://doi.org/10.1002/eco.2167, 2020.
Eager, C. D.: standarsize: Tools for Standardizing Variables for Regression in R, R package version 0.2.1, https://CRAN.R-project.org/package=standardize (last access: 20 October 2020), 2017.
Eggemeyer, K. D., Awada, T., Harvey, F. E., Wedin, D. A., Zhou, X., and Zanner, C. W.: Seasonal changes in depth of water uptake for encroaching trees Juniperus virginiana and Pinus ponderosa and two dominant C4 grasses in a semiarid grassland, Tree Physiol., 29, 157–169, https://doi.org/10.1093/treephys/tpn019, 2009.
Ehleringer, J. R. and Dawson, T. E.: Water uptake by plants: perspectives from stable isotope composition, Plant Cell Environ., 15, 1073–1082, https://doi.org/10.1111/j.1365-3040.1992.tb01657.x, 1992.
Ellsworth, P. Z. and Williams, D. G.: Hydrogen isotope fractionation during water uptake by woody xerophytes, Plant Soil, 291, 93–107, https://doi.org/10.1007/s11104-006-9177-1, 2007.
Esteban, G. J. and Robert, B. J.: The distribution of soil nutrients with depth: Global patterns and the imprint of plants, Biogeochemistry, 53, 51–77, https://doi.org/10.1023/A:1010760720215, 2001.
Estrada-Medina, H., Santiago, L. S., Graham, R. C., Allen, M. F., and Jiménez-Osornio, J. J.: Source water, phenology and growth of two tropical dry forest tree species growing on shallow karst soils, Trees – Struct. Funct., 27, 1297–1307, https://doi.org/10.1007/s00468-013-0878-9, 2013.
Evaristo, J. and McDonnell, J. J.: Prevalence and magnitude of groundwater use by vegetation: A global stable isotope meta-analysis, Sci. Rep.-UK, 7, 1–12, https://doi.org/10.1038/srep44110, 2017.
Evaristo, J., McDonnell, J. J., Scholl, M. A., Bruijnzeel, L. A., and Chun, K. P.: Insights into plant water uptake from xylem-water isotope measurements in two tropical catchments with contrasting moisture conditions, Hydrol. Process., 30, 3210–3227, https://doi.org/10.1002/hyp.10841, 2016.
FAO and UNESCO: Digital soil map of the world and derived soil properties, http://www.fao.org/land-water/land/land-governance/land-resources-planning-toolbox/category/details/en/c/1026564/ (last access: 20 October 2020), 2003.
Feikema, P. M., Morris, J. D., and Connell, L. D.: The water balance and water sources of a Eucalyptus plantation over shallow saline groundwater, Plant Soil, 332, 429–449, https://doi.org/10.1007/s11104-010-0309-2, 2010.
Flo, V., Martínez-Vilalta, J., Mencuccini, M., Granda, V., Anderegg, W. R. L., and Poyatos, R.: Climate and functional traits jointly mediate tree water-use strategies, New Phytol., 231, 617–630, https://doi.org/10.1111/nph.17404, 2021.
Gaines, K. P., Stanley, J. W., Meinzer, F. C., McCulloh, K. A., Woodruff, D. R., Chen, W., Adams, T. S., Lin, H., and Eissenstat, D. M.: Reliance on shallow soil water in a mixed-hardwood forest in central Pennsylvania, Tree Physiol., 36, 444–458, https://doi.org/10.1093/treephys/tpv113, 2016.
Geißler, K., Heblack, J., Uugulu, S., Wanke, H., and Blaum, N.: Partitioning of Water Between Differently Sized Shrubs and Potential Groundwater Recharge in a Semiarid Savanna in Namibia, Front. Plant Sci., 10, 1–13, https://doi.org/10.3389/fpls.2019.01411, 2019.
Geris, J., Tetzlaff, D., Mcdonnell, J., Anderson, J., Paton, G., and Soulsby, C.: Ecohydrological separation in wet, low energy northern environments? A preliminary assessment using different soil water extraction techniques, Hydrol. Process., 29, 5139–5152, https://doi.org/10.1002/hyp.10603, 2015.
Geris, J., Tetzlaff, D., McDonnell, J. J., and Soulsby, C.: Spatial and temporal patterns of soil water storage and vegetation water use in humid northern catchments, Sci. Total Environ., 595, 486–493, https://doi.org/10.1016/j.scitotenv.2017.03.275, 2017.
Gierke, C., Newton, B. T., and Phillips, F. M.: Interaction sol-eau et prélèvement d'eau par les arbres dans les montagnes de Sacramento dans le Nouveau Mexique (Etats-Unis d'Amérique): une étude des isotopes stables, Hydrogeol. J., 24, 805–818, https://doi.org/10.1007/s10040-016-1403-1, 2016.
Gómez-Navarro, C., Pataki, D. E., Bowen, G. J., and Oerter, E. J.: Spatiotemporal variability in water sources of urban soils and trees in the semiarid, irrigated Salt Lake Valley, Ecohydrology, 12, e2154, https://doi.org/10.1002/eco.2154, 2019.
Grossiord, C., Sevanto, S., Dawson, T. E., Adams, H. D., Collins, A. D., Dickman, L. T., Newman, B. D., Stockton, E. A., and McDowell, N. G.: Warming combined with more extreme precipitation regimes modifies the water sources used by trees, New Phytol., 213, 584–596, https://doi.org/10.1111/nph.14192, 2017.
Guo, F., Ma, J. J., Zheng, L. J., Sun, X. H., Guo, X. H., and Zhang, X. L.: Estimating distribution of water uptake with depth of winter wheat by hydrogen and oxygen stable isotopes under different irrigation depths, J. Integr. Agric., 15, 891–906, https://doi.org/10.1016/S2095-3119(15)61258-8, 2016.
Hartsough, P., Poulson, S. R., Biondi, F., and Estrada, I. G.: Stable isotope characterization of the ecohydrological cycle at a tropical treeline site, Arctic, Antarct. Alp. Res., 40, 343–354, https://doi.org/10.1657/1523-0430(06-117)[HARTSOUGH]2.0.CO;2, 2008.
Hersbach, H., Bell, B., Berrisford, P., Biavati, G., Horányi, A., Muñoz Sabater, J., Nicolas, J., Peubey, C., Radu, R., Rozum, I., Schepers, D., Simmons, A., Soci, C., Dee, D., and Thépaut, J.-N.: ERA5 monthly averaged data on single levels from 1979 to present, Copernicus Climate Change Service (C3S) Climate Data Store (CDS) [data set], https://doi.org/10.24381/cds.f17050d7, 2019.
Hervé-Fernández, P., Oyarzún, C., Brumbt, C., Huygens, D., Bodé, S., Verhoest, N. E. C., and Boeckx, P.: Assessing the `two water worlds' hypothesis and water sources for native and exotic evergreen species in south-central Chile, Hydrol. Process., 30, 4227–4241, https://doi.org/10.1002/hyp.10984, 2016.
Higgins, J. P. T. and Thompson, S. G.: Quantifying heterogeneity in a meta-analysis, Stat. Med., 21, 1539–1558, https://doi.org/10.1002/sim.1186, 2002.
Holland, K. L., Tyerman, S. D., Mensforth, L. J., and Walker, G. R.: Tree water sources over shallow, saline groundwater in the lower River Murray, south-eastern Australia: Implications for groundwater recharge mechanisms, Aust. J. Bot., 54, 193–205, https://doi.org/12750.1071/BT05019, 2006.
Huang, L. and Zhang, Z.: Stable Isotopic Analysis on Water Utilization of Two Xerophytic Shrubs in a Revegetated Desert Area: Tengger Desert, China, Water, 7, 1030–1045, https://doi.org/10.3390/w7031030, 2015.
IAEA/WMO: Global Network of Isotopes in Precipitation, The GNIP Database, https://nucleus.iaea.org/wiser (last access: 20 October 2020), 2015.
Illuminati, A., Querejeta, J., Pías, B., Escudero, A., and Matesanz, S.: Coordination between water uptake depth and the leaf economic spectrum in a Mediterranuean shrubland, J. Ecol., https://doi.org/10.1111/1365-2745.13909, in press, 2022.
Jespersen, R. G., Leffler, A. J., Oberbauer, S. F., and Welker, J. M.: Arctic plant ecophysiology and water source utilization in response to altered snow: isotopic (δ18O and δ2H) evidence for meltwater subsidies to deciduous shrubs, Oecologia, 187, 1009–1023, https://doi.org/10.1007/s00442-018-4196-1, 2018.
Jia, D. J., Qian, L., and Jian, J.: The seasonal water use patterns of populus pseudo-simmonii Kitag in the Otindag Sandy Land, Fresenius Environ. Bull., 27, 4037–4046, 2018.
Jiménez-Rodríguez, C. D., Coenders-Gerrits, M., Uhlenbrook, S., and Wenninger, J.: What do plants leave after summer on the ground?-The effect of afforested plants in arid environments, Water, 11, 2559, https://doi.org/10.3390/w11122559, 2019.
Johnson, D. M., McCulloh, K. A., Woodruff, D. R., and Meinzer, F. C.: Hydraulic safety margins and embolism reversal in stems and leaves: Why are conifers and angiosperms so different?, Plant Sci., 195, 48–53, https://doi.org/10.1016/j.plantsci.2012.06.010, 2012.
Jones, C., Stanton, D., Hamer, N., Denner, S., Singh, K., Flook, S., and Dyring, M.: Field investigation of potential terrestrial groundwater-dependent ecosystems within Australia's Great Artesian Basin, Hydrogeol. J., 28, 237–261, https://doi.org/10.1007/s10040-019-02081-1, 2020.
Knighton, J., Kuppel, S., Smith, A., Soulsby, C., Sprenger, M., and Tetzlaff, D.: Using isotopes to incorporate tree water storage and mixing dynamics into a distributed ecohydrologic modelling framework, Ecohydrology, 13, e2201, https://doi.org/10.1002/eco.2201, 2020.
Koricheva, J., Gurevitch, J., and Mengersen, K. (Eds.): Handbook of Meta-Analysis In Ecology and Evolution, Princeton University Press, ISBN 9780691137285, 2013.
Kulmatiski, A., Beard, K. H., and Stark, J. M.: Exotic plant communities shift water-use timing in a shrub-steppe ecosystem, Plant Soil, 288, 271–284, https://doi.org/10.1007/s11104-006-9115-2, 2006.
Kuznetsova, A., Brockhoff, P. B., and Christensen, R. H. B.: lmerTest Package: Tests in Linear Mixed Effects Models, J. Stat. Softw., 82, 1–26, https://doi.org/10.18637/jss.v082.i13, 2017.
Landwehr, J. M. and Coplen, T. B.: Line-conditioned excess: a new method for characterizing stable hydrogen and oxygen isotope ratios in hydrologic systems, Int. Conf. Isot. Environ. Stud., 37, 132–135, 2006.
Leng, X., Cui, J., Zhang, S., Zhang, W., Liu, Y., Liu, S., and An, S.: Differential water uptake among plant species in humid alpine meadows, J. Veg. Sci., 24, 138–147, https://doi.org/10.1111/j.1654-1103.2012.01439.x, 2013.
Li, X., Wigneron, J. P., Frappart, F., Fan, L., Ciais, P., Fensholt, R., Entekhabi, D., Brandt, M., Konings, A. G., Liu, X., Wang, M., Al-Yaari, A., and Moisy, C.: Global-scale assessment and inter-comparison of recently developed/reprocessed microwave satellite vegetation optical depth products, Remote Sens. Environ., 253, 112208, https://doi.org/10.1016/j.rse.2020.112208, 2021.
Lin, G. and Sternberg, L.: Hydrogen Isotopic Fractionation by Plant Roots during Water Uptake in Coastal Wetland Plants, edited by: Ehleringer, J. R., Hall, A. E., and Farquhar, G. D., Academic Press, Inc., https://doi.org/10.1016/B978-0-08-091801-3.50041-6, 1993.
Liu, J., Shen, L., Wang, Z., Duan, S., Wu, W., Peng, X., Wu, C., and Jiang, Y.: Response of plants water uptake patterns to tunnels excavation based on stable isotopes in a karst trough valley, J. Hydrol., 571, 485–493, https://doi.org/10.1016/j.jhydrol.2019.01.073, 2019.
Liu, W., Li, P., Duan, W., and Liu, W.: Dry-season water utilization by trees growing on thin karst soils in a seasonal tropical rainforest of Xishuangbanna, Southwest China, Ecohydrology, 7, e1419, https://doi.org/10.1002/eco.1419, 2014.
Liu, W., Jia, J., Yu, X., and Liu, Z.: Water Sources of the Oak-pine Mixed Community in Beijing Mountainous Area, Yingyong Jichu yu Gongcheng Kexue Xuebao/Journal Basic Sci. Eng., 26, 12–22, https://doi.org/10.16058/j.issn.1005-0930.2018.01.002, 2018.
Liu, Y., Xu, Z., Duffy, R., Chen, W., An, S., Liu, S., and Liu, F.: Analyzing relationships among water uptake patterns, rootlet biomass distribution and soil water content profile in a subalpine shrubland using water isotopes, Eur. J. Soil Biol., 47, 380–386, https://doi.org/10.1016/j.ejsobi.2011.07.012, 2011.
Li, Y., Ma, Y., Song, X., Wang, L., and Han, D.: A δ2H offset correction method for quantifying root water uptake of riparian trees, J. Hydrol., 593, 125811, https://doi.org/10.1016/j.jhydrol.2020.125811, 2020.
Liu, Z., Yu, X., and Jia, G.: Water uptake by coniferous and broad-leaved forest in a rocky mountainous area of northern China, Agr. Forest Meteorol., 265, 381–389, https://doi.org/10.1016/j.agrformet.2018.11.036, 2019a.
Liu, Z., Yu, X., and Jia, G.: Water uptake by coniferous and broad-leaved forest in a rocky mountainous area of northern China, Agr. Forest Meteorol., 265, 381–389, https://doi.org/10.1016/j.agrformet.2018.11.036, 2019b.
Liu, Z., Jia, G., and Yu, X.: Variation of water uptake in degradation agroforestry shelterbelts on the North China Plain, Agric. Ecosyst. Environ., 287, 106697, https://doi.org/10.1016/j.agee.2019.106697, 2020.
Lovelock, C. E., Reef, R., and Ball, M. C.: Isotopic signatures of stem water reveal differences in water sources accessed by mangrove tree species, Hydrobiologia, 803, 133–145, https://doi.org/10.1007/s10750-017-3149-8, 2017.
Lüdecke, D., Ben-Shachar, M., Patil, I., Waggoner, W., and Makowski, M.: performance: An R Package for Assessment, Comparison and Testing of Statistical Models, J. Open Source Softw., 6, 3139, https://doi.org/10.21105/joss.03139, 2020.
Luo, Z., Guan, H., Zhang, X., Xu, X., Dai, J., and Hua, M.: Examination of the ecohydrological separation hypothesis in a humid subtropical area: Comparison of three methods, J. Hydrol., 571, 642–650, https://doi.org/10.1016/j.jhydrol.2019.02.019, 2019.
Ma, Y. and Song, X.: Using stable isotopes to determine seasonal variations in water uptake of summer maize under different fertilization treatments, Sci. Total Environ., 550, 471–483, https://doi.org/10.1016/j.scitotenv.2016.01.148, 2016.
Magh, R. K., Eiferle, C., Burzlaff, T., Dannenmann, M., Rennenberg, H., and Dubbert, M.: Competition for water rather than facilitation in mixed beech-fir forests after drying-wetting cycle, J. Hydrol., 587, 124944, https://doi.org/10.1016/j.jhydrol.2020.124944, 2020.
Maherali, H., Oberle, B., Stevens, P. F., Cornwell, W. K., and McGlinn, D. J.: Mutualism persistence and abandonment during the evolution of the mycorrhizal symbiosis, Am. Nat., 188, E113–E125, https://doi.org/10.1086/688675, 2016.
Mamonov, A. B., Coalson, R. D., Zeidel, M. L., and Mathai, J. C.: Water and deuterium oxide permeability through aquaporin 1: MD predictions and experimental verification, J. Gen. Physiol., 130, 111–116, https://doi.org/10.1085/jgp.200709810, 2007.
Marshall, J. D., Cuntz, M., Beyer, M., Dubbert, M., and Kuehnhammer, K.: Borehole Equilibration: Testing a New Method to Monitor the Isotopic Composition of Tree Xylem Water in situ, Front. Plant Sci., 11, 1–14, https://doi.org/10.3389/fpls.2020.00358, 2020.
Marttila, H., Dudley, B. D., Graham, S., and Srinivasan, M. S.: Does transpiration from invasive stream side willows dominate low-flow conditions? An investigation using hydrometric and isotopic methods in a headwater catchment, Ecohydrology, 11, e1930, https://doi.org/10.1002/eco.1930, 2018.
Martín-Gómez, P., Barbeta, A., Voltas, J., Peñuelas, J., Dennis, K., Palacio, S., Dawson, T. E., and Ferrio, J. P.: Isotope-ratio infrared spectroscopy: A reliable tool for the investigation of plant-water sources?, New Phytol., 207, 914–927, https://doi.org/10.1111/nph.13376, 2015.
Martín-Gómez, P., Serrano, L., and Ferrio, J. P.: Short-term dynamics of evaporative enrichment of xylem water in woody stems: Implications for ecohydrology, Tree Physiol., 37, 511–522, https://doi.org/10.1093/treephys/tpw115, 2017.
Martinez-Vilalta, J., Anderegg, W. R. L., Sapes, G., and Sala, A.: Greater focus on water pools may improve our ability to understand and anticipate drought-induced mortality in plants, New Phytol., 223, 22–32, https://doi.org/10.1111/nph.15644, 2019.
McCole, A. A. and Stern, L. A.: Seasonal water use patterns of Juniperus ashei on the Edwards Plateau, Texas, based on stable isotopes in water, J. Hydrol., 342, 238–248, https://doi.org/10.1016/j.jhydrol.2007.05.024, 2007.
McCutcheon, R. J., McNamara, J. P., Kohn, M. J., and Evans, S. L.: An evaluation of the ecohydrological separation hypothesis in a semiarid catchment, Hydrol. Process., 31, 783–799, https://doi.org/10.1002/hyp.11052, 2017.
McKeon, C., Glenn, E. P., Waugh, W. J., Eastoe, C., Jordan, F., and Nelson, S. G.: Growth and water and nitrate uptake patterns of grazed and ungrazed desert shrubs growing over a nitrate contamination plume, J. Arid Environ., 64, 1–21, https://doi.org/10.1016/j.jaridenv.2005.04.008, 2006.
Mensforth, L. J., Thorburn, P. J., Tyerman, S. D., and Walker, G. R.: Sources of water used by riparian Eucalyptus camaldulensis overlying highly saline groundwater, Oecologia, 100, 21–28, https://doi.org/10.1007/BF00317126, 1994.
Miller, G. R., Chen, X., Rubin, Y., Ma, S., and Baldocchi, D. D.: Groundwater uptake by woody vegetation in a semiarid oak savanna, Water Resour. Res., 46, W10503, https://doi.org/10.1029/2009WR008902, 2010.
Moore, G., Li, F., Kui, L., and West, J.: Flood water legacy as a persistent source for riparian vegetation during prolonged drought: an isotopic study of Arundo donax on the Rio Grande, Ecohydrology, 9, 909–917, https://doi.org/10.1002/eco.1698, 2016.
Morris, H., Gillingham, M. A. F., Plavcová, L., Gleason, S. M., Olson, M. E., Coomes, D. A., Fichtler, E., Klepsch, M. M., Martínez-Cabrera, H. I., McGlinn, D. J., Wheeler, E. A., Zheng, J., Ziemiñska, K., and Jansen, S.: Vessel diameter is related to amount and spatial arrangement of axial parenchyma in woody angiosperms, Plant Cell Environ., 41, 245–260, https://doi.org/10.1111/pce.13091, 2018.
Muñoz-Villers, L. E., Holwerda, F., Alvarado-Barrientos, M. S., Geissert, D. R., and Dawson, T. E.: Reduced dry season transpiration is coupled with shallow soil water use in tropical montane forest trees, Oecologia, 188, 303–317, https://doi.org/10.1007/s00442-018-4209-0, 2018.
Muñoz-Villers, L. E., Geris, J., Alvarado-Barrientos, M. S., Holwerda, F., and Dawson, T.: Coffee and shade trees show complementary use of soil water in a traditional agroforestry ecosystem, Hydrol. Earth Syst. Sci., 24, 1649–1668, https://doi.org/10.5194/hess-24-1649-2020, 2020.
Nehemy, M. F., Benettin, P., Asadollahi, M., Pratt, D., Rinaldo, A., and McDonnell, J. J.: Tree water deficit and dynamic source water partitioning, Hydrol. Process., 35, e14004, https://doi.org/10.1002/hyp.14004, 2020.
Newberry, S. L., Nelson, D. B., and Kahmen, A.: Cryogenic vacuum artifacts do not affect plant water-uptake studies using stable isotope analysis, Ecohydrology, 10, e1892, https://doi.org/10.1002/eco.1892, 2017.
Nie, Y., Chen, H., Wang, K., Tan, W., Deng, P., and Yang, J.: Seasonal water use patterns of woody species growing on the continuous dolostone outcrops and nearby thin soils in subtropical China, Plant Soil, 341, 399–412, https://doi.org/10.1007/s11104-010-0653-2, 2011.
Ohte, N., Koba, K., Yoshikawa, K., Sugimoto, A., Matsuo, N., Kabeya, N., and Wang, L.: Water utilization of natural and planted trees in the semiarid desert of Inner Mongolia, China, Ecol. Appl., 13, 337–351, https://doi.org/10.1890/1051-0761(2003)013[0337:WUONAP]2.0.CO;2, 2003.
Orlowski, N., Breuer, L., Angeli, N., Boeckx, P., Brumbt, C., Cook, C. S., Dubbert, M., Dyckmans, J., Gallagher, B., Gralher, B., Herbstritt, B., Hervé-Fernández, P., Hissler, C., Koeniger, P., Legout, A., Macdonald, C. J., Oyarzún, C., Redelstein, R., Seidler, C., Siegwolf, R., Stumpp, C., Thomsen, S., Weiler, M., Werner, C., and McDonnell, J. J.: Inter-laboratory comparison of cryogenic water extraction systems for stable isotope analysis of soil water, Hydrol. Earth Syst. Sci., 22, 3619–3637, https://doi.org/10.5194/hess-22-3619-2018, 2018.
Poca, M., Coomans, O., Urcelay, C., Zeballos, S. R., Bodé, S., and Boeckx, P.: Isotope fractionation during root water uptake by Acacia caven is enhanced by arbuscular mycorrhizas, Plant Soil, 441, 485–497, https://doi.org/10.1007/s11104-019-04139-1, 2019.
Qian, J., Zheng, H., Wang, P., Liao, X., Wang, C., Hou, J., Ao, Y., Shen, M., Liu, J., and Li, K.: Assessing the ecohydrological separation hypothesis and seasonal variations in water use by Ginkgo biloba L. in a subtropical riparian area, J. Hydrol., 553, 486–500, https://doi.org/10.1016/j.jhydrol.2017.08.021, 2017a.
Qian, J., Zheng, H., Wang, P., Liao, X., Wang, C., Li, K., Liu, J., Lu, B., Tian, X., and Yuan, W.: Water sources of riparian plants during a rainy season in Taihu Lake Basin, China: A stable isotope study, Chem. Speciat. Bioavail., 29, 153–160, https://doi.org/10.1080/09542299.2017.1373035, 2017b.
R Core Team: R: A language and environment for statistical computing, https://www.r-project.org, last access: 20 October 2020.
Ripullone, F., Camarero, J. J., Colangelo, M. ,and Voltas, J.: Variation in the access to deep soil water pools explains tree-to-tree differences in drought-triggered dieback of Mediterranean oaks, Tree Physiol., 40, 591–604, https://doi.org/10.1093/treephys/tpaa026, 2020.
Rohatgi, A.: WebPlotDigitizer, https://automeris.io/WebPlotDigitizer, last access: 20 October 2020.
Rong, L., Chen, X., Chen, X., Wang, S., and Du, X.: Isotopic analysis of water sources of mountainous plant uptake in a karst plateau of southwest China, Hydrol. Process., 25, 3666–3675, https://doi.org/10.1002/hyp.8093, 2011.
Rose, K. L., Graham, R. C., and Parker, D. R.: Water source utilization by Pinus jeffreyi and Arctostaphylos patula on thin soils over bedrock, Oecologia, 134, 46–54, https://doi.org/10.1007/s00442-002-1084-4, 2003.
Rossatto, D. R., de Carvalho Ramos Silva, L., Villalobos-Vega, R., da Silveira Lobo Sternberg, L., and Franco, A. C.: Depth of water uptake in woody plants relates to groundwater level and vegetation structure along a topographic gradient in a neotropical savanna, Environ. Exp. Bot., 77, 259–266, https://doi.org/10.1016/j.envexpbot.2011.11.025, 2012.
Saha, S., Sadle, J., Van Der Heiden, C., and Sternberg, L.: Salinity, groundwater, and water uptake depth of plants in coastal uplands of everglades national park (florida, USA), Ecohydrology, 8, 128–136, https://doi.org/10.1002/eco.1494, 2015.
Sapes, G. and Sala, A.: Relative water content consistently predicts drought mortality risk in seedling populations with different morphology, physiology and times to death, Plant Cell Environ., 44, 3322–3335, https://doi.org/10.1111/pce.14149, 2021.
Schmidt, M., Maseyk, K., Lett, C., Biron, P., Richard, P., Bariac, T., and Seibt, U.: Reducing and correcting for contamination of ecosystem water stable isotopes measured by isotope ratio infrared spectroscopy, Rapid Commun. Mass Sp., 26, 141–153, https://doi.org/10.1002/rcm.5317, 2012.
Schulze, E. D., Mooney, H. A., Sala, O. E., Jobbagy, E., Buchmann, N., Bauer, G., Canadell, J., Jackson, R. B., Loreti, J., Oesterheld, M., and Ehleringer, J. R.: Rooting depth, water availability, and vegetation cover along an aridity gradient in Patagonia, Oecologia, 108, 503–511, https://doi.org/10.1007/BF00333727, 1996.
Schwendenmann, L.: Data: H and O isotope signatures of soil and xylem samples, cacao agroforest, figshare [data set], https://doi.org/10.17608/k6.auckland.8067449.v1, 2019.
Schwendenmann, L. and Jost, F.: Data: Water stable isotope signature of soil and xylem samples under different land use systems, figshare [data set], https://doi.org/10.17608/k6.auckland.8067338.v1, 2019.
Schwendenmann, L., Pendall, E., Sanchez-Bragado, R., Kunert, N., and Hölscher, D.: Tree water uptake in a tropical plantation varying in tree diversity: Interspecific differences, seasonal shifts and complementarity, Ecohydrology, 8, 1–12, https://doi.org/10.1002/eco.1479, 2015.
Searle, S. R., Speed, F. M., and Milliken, G. A.: Population marginal means in the linear model: An alternative to least squares means, Am. Stat., 34, 216–221, https://doi.org/10.1080/00031305.1980.10483031, 1980.
Simonin, K. A., Link, P., Rempe, D., Miller, S., Oshun, J., Bode, C., Dietrich, W. E., Fung, I., and Dawson, T. E.: Vegetation induced changes in the stable isotope composition of near surface humidity, Ecohydrology, 7, 936–949, https://doi.org/10.1002/eco.1420, 2014.
Snelgrove, J. R., Buttle, J. M., Kohn, M. J., and Tetzlaff, D.: Co-evolution of xylem water and soil water stable isotopic composition in a northern mixed forest biome, Hydrol. Earth Syst. Sci., 25, 2169–2186, https://doi.org/10.5194/hess-25-2169-2021, 2021.
Snyder, K. A. and Williams, D. G.: Water sources used by riparian trees varies among stream types on the San Pedro River, Arizona, Agr. Forest Meteorol., 105, 227–240, https://doi.org/10.1016/S0168-1923(00)00193-3, 2000.
Song, L., Zhu, J., Li, M., and Yu, Z.: Water utilization of pinus sylvestris var. mongolica in a sparse wood grassland in the semiarid sandy region of Northeast China, Trees – Struct. Funct., 28, 971–982, https://doi.org/10.1007/s00468-014-1010-5, 2014.
Song, L., Zhu, J., Li, M., Zhang, J., and Lv, L.: Sources of water used by Pinus sylvestris var. mongolica trees based on stable isotope measurements in a semiarid sandy region of Northeast China, Agr. Water Manage., 164, 281–290, https://doi.org/10.1016/j.agwat.2015.10.018, 2016.
Sprenger, M., Leistert, H., Gimbel, K., and Weiler, M.: Illuminating hydrological processes at the soil-vegetation-atmosphere interface with water stable isotopes, Rev. Geophys., 54, 674–704, https://doi.org/10.1002/2015RG000515, 2016.
Stock, B. C., Jackson, A. L., Ward, E. J., Parnell, A. C., Phillips, D. L., and Semmens, B. X.: Analyzing mixing systems using a new generation of Bayesian tracer mixing models, PeerJ, 2018, 1–27, https://doi.org/10.7717/peerj.5096, 2018.
Su, P., Zhang, M., Qu, D., Wang, J., Zhang, Y., Yao, X., and Xiao, H.: Contrasting water use strategies of tamarix ramosissima in different habitats in the northwest of loess plateau, china, Water (Switzerland), 12, 8–15, https://doi.org/10.3390/w12102791, 2020.
Sun, L., Yang, L., Chen, L., Zhao, F., and Li, S.: Short-term changing patterns of stem water isotopes in shallow soils underlain by fractured bedrock, Hydrol. Res., 50, 577–588, https://doi.org/10.2166/nh.2018.086, 2019.
Swaffer, B. A., Holland, K. L., Doody, T. M., Li, C., and Hutson, J.: Water use strategies of two co-occurring tree species in a semi-arid karst environment, Hydrol. Process., 28, 2003–2017, https://doi.org/10.1002/hyp.9739, 2014.
Taylor, J. R.: An introduction to error analysis; the study of uncertainties in physical measurements, 2nd Edn., University Science Books, Sausalito, ISBN 9780935702750, 1997.
Tetzlaff, D., Buttle, J., Carey, S. K., Kohn, M. J., Laudon, H., McNamara, J. P., Smith, A., Sprenger, M., and Soulsby, C.: Stable isotopes of water reveal differences in plant – soil water relationships across northern environments, Hydrol. Process., 35, 1–19, https://doi.org/10.1002/hyp.14023, 2021.
Thorburn, P. J., Walker, G. R., and Jolly, I. D.: Uptake of saline groundwater by plants: An analytical model for semi-arid and arid areas, Plant Soil, 175, 1–11, https://doi.org/10.1007/BF02413005, 1995.
Twining, J., Stone, D., Tadros, C., Henderson-Sellers, A., and Williams, A.: Moisture Isotopes in the Biosphere and Atmosphere (MIBA) in Australia: A priori estimates and preliminary observations of stable water isotopes in soil, plant and vapour for the Tumbarumba Field Campaign, Global Planet. Change, 51, 59–72, https://doi.org/10.1016/j.gloplacha.2005.12.005, 2006.
Vargas, A. I., Schaffer, B., Yuhong, L., and Sternberg, L. d. S. L.: Testing plant use of mobile vs immobile soil water sources using stable isotope experiments, New Phytol., 215, 582–594, https://doi.org/10.1111/nph.14616, 2017.
Voltas, J., Lucabaugh, D., Chambel, M. R., and Ferrio, J. P.: Voltas_Intraspecific variation in the use of water sources bythe circum-Mediterranean conifer Pinus halepensis, New Phytol., 208, 1031–1041, https://doi.org/10.1111/nph.13569, 2015.
Wang, J., Fu, B., Lu, N., and Zhang, L.: Seasonal variation in water uptake patterns of three plant species based on stable isotopes in the semi-arid Loess Plateau, Sci. Total Environ., 609, 27–37, https://doi.org/10.1016/j.scitotenv.2017.07.133, 2017.
Wang, J., Lu, N., and Fu, B.: Inter-comparison of stable isotope mixing models for determining plant water source partitioning, Sci. Total Environ., 666, 685–693, https://doi.org/10.1016/j.scitotenv.2019.02.262, 2019.
Washburn, E. W. and Smith, E. R.: The isotopic fractionation of water by physiological processes, Science, 79, 188–189, 1934.
Wei, Y. F., Fang, J., Liu, S., Zhao, X. Y., and Li, S. G.: Stable isotopic observation of water use sources of Pinus sylvestris var. mongolica in Horqin Sandy Land, China, Trees – Struct. Funct., 27, 1249–1260, https://doi.org/10.1007/s00468-013-0873-1, 2013.
West, A. G., Patrickson, S. J., and Ehleringer, J. R.: Water extraction times for plant and soil materials used in stable isotope analysis, Rapid Commun. Mass Sp., 20, 1817–1321, https://doi.org/10.1002/rcm.2456, 2006.
West, A. G., Hultine, K. R., Burtch, K. G., and Ehleringer, J. R.: Seasonal variations in moisture use in a piñon-juniper woodland, Oecologia, 153, 787–798, https://doi.org/10.1007/s00442-007-0777-0, 2007.
West, A. G., Goldsmith, G. R., Brooks, P. D., and Dawson, T. E.: Discrepancies between isotope ratio infrared spectroscopy and isotope ratio mass spectrometry for the stable isotope analysis of plant and soil waters, Rapid Commun. Mass Sp., 24, 1457–1466, https://doi.org/10.1002/rcm.4597, 2010.
Wu, H., Li, X. Y., Jiang, Z., Chen, H., Zhang, C., and Xiao, X.: Contrasting water use pattern of introduced and native plants in an alpine desert ecosystem, Northeast Qinghai-Tibet Plateau, China, Sci. Total Environ., 542, 182–191, https://doi.org/10.1016/j.scitotenv.2015.10.121, 2016a.
Wu, H., Li, X. Y., Li, J., Jiang, Z., Chen, H., Ma, Y., and Huang, Y.: Differential soil moisture pulse uptake by coexisting plants in an alpine Achnatherum splendens grassland community, Environ. Earth Sci., 75, 914, https://doi.org/10.1007/s12665-016-5694-2, 2016b.
Wu, H., Li, J., Zhang, C., He, B., Zhang, H., Wu, X., and Li, X.-Y.: Determining root water uptake of two alpine crops in a rainfed cropland in the Qinghai Lake watershed: First assessment using stable isotopes analysis, Field Crop. Res., 215, 113–121, https://doi.org/10.1016/j.fcr.2017.10.011, 2018.
Yang, B., Wen, X., and Sun, X.: Seasonal variations in depth of water uptake for a subtropical coniferous plantation subjected to drought in an East Asian monsoon region, Agr. Forest Meteorol., 201, 218–228, https://doi.org/10.1016/j.agrformet.2014.11.020, 2015.
Yin, L., Zhou, Y., Huang, J., Wenninger, J., Zhang, E., Hou, G., and Dong, J.: Interaction between groundwater and trees in an arid site: Potential impacts of climate variation and groundwater abstraction on trees, J. Hydrol., 528, 435–448, https://doi.org/10.1016/j.jhydrol.2015.06.063, 2015.
Zhang, C., Zhang, J., Zhao, B., Zhu, A., Zhang, H., Huang, P., and Li, X.: Coupling a two-tip linear mixing model with a δD–δ18O plot to determine water sources consumed by maize during different growth stages, Field Crop. Res., 123, 196–205, https://doi.org/10.1016/j.fcr.2011.04.018, 2011.
Zhao, L., Wang, L., Cernusak, L. A., Liu, X., Xiao, H., Zhou, M., and Zhang, S.: Significant Difference in Hydrogen Isotope Composition Between Xylem and Tissue Water in Populus Euphratica, Plant Cell Environ., 39, 1848–1857, https://doi.org/10.1111/pce.12753, 2016.
Zhao, L.-J., Wang, X.-G., Zhang, Y.-C., Xie, C., Liu, Q.-Y. ,and Meng, F.: Plant water use strategies in the Shapotou artificial sand-fixed vegetation of the southeastern margin of the Tengger Desert, northwestern China, J. Mt. Sci., 16, 898–908, https://doi.org/10.1007/s11629-018-5028-9, 2019.
Zhao, P., Cornelis, W., Tang, X., Zhao, P., and Tang, J.: Does damming streams alter the water use strategies of riparian trees? A case study in a subtropic climate, L. Degrad. Dev., 31, 927–938, https://doi.org/10.1002/ldr.3500, 2020.
Zhao, W., Han, Y. Wei, X., and Liang, W.: Water uptake strategies by typical broadleaf and coniferous trees in the Loess Plateau mountain area of northern China, Appl. Ecol. Environ. Res., 19, 3273–3292, https://doi.org/10.15666/aeer/1904_32733292, 2021.
Zhou, H., Zhao, W., He, Z., Yan, J., and Zhang, G.: Variation in depth of water uptake for Pinus sylvestris var. mongolica along a precipitation gradient in sandy regions, J. Hydrol., 577, 123921, https://doi.org/10.1016/j.jhydrol.2019.123921, 2019.
Zhu, J., Liu, J., Lu, Z., Xia, J., Sun, J., Shao, H., and Zhao, Y.: Soil-water interacting use patterns driven by Ziziphus jujuba on the Chenier Island in the Yellow River Delta, China, Arch. Agron. Soil Sci., 62, 1614–1624, https://doi.org/10.1080/03650340.2016.1155702, 2016a.
Zhu, L., Zhang, H., Gao, X., Qi, Y., and Xu, X.: Seasonal patterns in water uptake for Medicago sativa grown along an elevation gradient with shallow groundwater table in Yanchi county of Ningxia, Northwest China, J. Arid Land, 8, 921–934, https://doi.org/10.1007/s40333-016-0017-8, 2016b.
Zimmerman, U., Ehhalt, D., and Munnich, K. O.: Soil Water Movement and Evapotranspiration: Changes in the Isotopic Composition of the Water, in: Isotopes in Hydrology, Proceedings of the Symposium, International Atomic Energy Agency (I.A.E.A.), Vienna, 567–584, 1967.
Zuecco, G., Amin, A., Frentress, J., Engel, M., Marchina, C., Anfodillo, T., Borga, M., Carraro, V., Scandellari, F., Tagliavini, M., Zanotelli, D., Comiti, F. and Penna, D.: A comparative study of plant water extraction methods for isotopic analyses: Scholander-type pressure chamber vs. cryogenic vacuum distillation, Hydrol. Earth Syst. Sci., 26), 3673–3689, https://doi.org/10.5194/hess-2020-446, 2022.