the Creative Commons Attribution 4.0 License.
the Creative Commons Attribution 4.0 License.
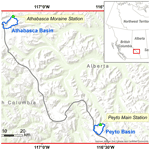
Recent hydrological response of glaciers in the Canadian Rockies to changing climate and glacier configuration
Dhiraj Pradhananga
John W. Pomeroy
Mountain snow and ice greatly influence the hydrological cycle of alpine regions by regulating both the quantity of and seasonal variations in water availability downstream. This study considers the combined impacts of climate and glacier changes due to recession on the hydrology and water balance of two high-elevation basins in the Canadian Rockies. A distributed, physically based, uncalibrated glacier hydrology model developed in the Cold Regions Hydrological Modelling platform (CRHM) was used to simulate the glacier mass balance and basin hydrology of the Peyto and Athabasca glacier basins in Alberta, Canada. Bias-corrected reanalysis data were used to drive the model. The model calculates the water balance of glacierized basins, influenced by the surface energy and mass balance, and considers the redistribution of snow by wind and avalanches. It was set up using hydrological response units based on elevation bands, surface slope, and aspect, as well as changing land cover. Aerial photos, satellite images and digital elevation models (DEMs) were assimilated to represent the changing configurations of glacier area and the exposure of ice and firn. Observations of glacier mass balance, snow, and glacier ice surface elevation changes at glacier and alpine tundra meteorological stations and streamflow discharge at the glacier outlets were used to evaluate the model performance. Basin hydrology was simulated over two periods, 1965–1975 and 2008–2018, using the observed glacier configurations for those time periods. Both basins have undergone continuous glacier loss over the last 3 to 5 decades, leading to a 6 %–31 % reduction in glacierized area, a 78 %–109 % increase in ice exposure, and changes to the elevation and slope of the glacier surfaces. Air temperatures are increasing, mainly due to increasing winter maximum and summer minimum daily temperatures. Annual precipitation has increased by less than 11 %, but rainfall ratios have increased by 29 %–44 %. The results show that changes in both climate and glacier configuration have influenced the melt rates and runoff and a shift of peak flows in the Peyto Glacier basin from August to July. Glacier melt contributions increased/decreased from 27 %–61 % to 43 %–59 % of the annual discharges. Recent discharges were 3 %–19 % higher than in the 1960s and 1970s. The results suggest that increased exposure of glacier ice and lower surface elevation due to glacier thinning were less influential than climate warming in increasing streamflow. Streamflow from these glaciers continues to increase.
- Article
(3902 KB) - Full-text XML
-
Supplement
(180 KB) - BibTeX
- EndNote
Mountain streamflow profoundly affects the quantity, quality, and seasonal variation in water in major downstream river systems, particularly in the arid and semiarid regions of western North America (Marks et al., 2008). Glaciers contribute significantly to this streamflow during warm and dry periods and, in doing so, moderate interannual variability and contribute to flow water during extreme warm and dry periods (Comeau et al., 2009; Fountain and Tangborn, 1985; Hopkinson and Young, 1998). North American mountain glaciers began to retreat after the Little Ice Age ended in the early 1800s (Barry, 2006; Riedel et al., 2015). In addition to warming after the Little Ice Age, western Canada has experienced a warming climate in recent decades, with changes in precipitation regimes and a decline in snow cover (DeBeer et al., 2016). As a result, glaciers in the region are retreating more rapidly than in the past (DeBeer et al., 2016; Schiefer et al., 2007; Tennant et al., 2012), and additional glacier meltwater is contributing to headwater streamflow discharge and groundwater recharge (Castellazzi et al., 2019).
Marshall et al. (2011) projected glacier volumes of the Canadian Rockies (eastern slopes) for the next century. Their projected values are alarming, as they indicate a further ∼ 85 % loss of glacier volume by 2100 and an order of magnitude decrease in glacier contribution to streamflow in Alberta from 1.1 km3 yr−1 at present to 0.1 km3 yr−1 at the end of this century. Similarly, Clarke et al. (2015) projected a 75 % loss of glacier mass in western Canada by the end of the 21st century compared to 2005.
It has been proposed that, as the climate warms, flow originating from glaciers will increase for a certain time, due to increased melt rates, and then decline as the glacier-covered area decreases (Moore et al., 2009). The duration and timing of this change from increasing to decreasing flow, however, will be regionally dependent on basin elevation and/or glacier coverage of the basin (Casassa et al., 2009). Stahl and Moore (2006) observed that late summer streamflow from many British Columbia glacierized mountain basins has been declining, which suggests that most source glaciers have already completed the phase of increased flow due to rising temperatures and increasing melt rates and now contribute less streamflow as their areas decline. Chernos et al. (2020) projected a rise in glacier discharge in the Athabasca River basin in Alberta until the mid-21st century and then reduced discharge. Similarly, Neupane et al. (2018) modelled the upper Athabasca River basin and assessed the effects of changes in temperature and precipitation on simulated future discharge for the 2080–2099 period. They projected a reduction in water availability in the basin during the summer months.
Glacier mass loss is associated with a reduction in the glacier-covered area, an increase in ice exposure compared to that of snow and firn, and changes to the elevation and slope of the glacier surface. However, it is yet to be fully understood how the changes in glacier configuration and climate impact the streamflow jointly and individually. The integrated impacts of climate change on mountain streamflow are complex, and sometimes changes in hydrological processes can have compensating effects on streamflow generation under a changing climate, e.g., a reduction in one contribution is compensated for by an increase in another contribution (Fang and Pomeroy, 2020; Harder et al., 2015). Therefore, the impacts from climate change and glacier change on hydrology need to be diagnosed both separately and together. This study investigates the individual and combined impacts of changing climate and receding glaciers on the headwater hydrology of two well-instrumented glacierized basins on the eastern slopes of the Canadian Rockies, using a cold regions glacier hydrological model, CRHM-glacier (Cold Regions Hydrological Model – Glacier) forced by bias-corrected reanalysis data.
2.1 Study basins
In total, two alpine glacier basins in the Canadian Rockies, Alberta, Canada (Fig. 1), were chosen for this research, namely the Peyto Glacier research basin (PGRB; 22.43 km2) in Banff National Park and Athabasca Glacier research basin (AGRB; 29.3 km2) in Jasper National Park. The details of these basins are provided by Pradhananga and Pomeroy (2022) and Pradhananga et al. (2021). Both glaciers are instrumented with on-ice meteorological stations on the lower ice tongues of the glacier and off-ice meteorological stations on moraine below the tongue, and their outlet streams are gauged at the outlets of their current proglacial lakes. Both glaciers have been losing mass continuously since the mid-1970s (Demuth and Keller, 2006; Intsiful and Ambinakudige, 2021; Kehrl et al., 2014; Reynolds and Young, 1997; Tennant and Menounos, 2013). Clarke et al. (2015) projected that AGRB will lose half of its glacier coverage by 2050. Kehrl et al. (2014) estimated that Peyto Glacier may lose about 85 % of its present-day mass by 2100.
2.2 Meteorological forcing datasets
Bias-corrected ERA-40 (Uppala et al., 2005) and ERA-Interim reanalysis data (Dee et al., 2011), representing surface levels for air temperature, vapour pressure, wind speed, precipitation, and incoming short- and longwave radiation were used to force the CRHM-glacier model. The ERA-40 was available for the period of 1957–2002 and ERA-Interim for the period of 1979–2019, with an overlapping period of 1979–2002. These ERA global reanalyses were first bias corrected to in situ observational datasets from meteorological stations near to the glaciers (Athabasca Moraine Station for AGRB and Peyto Main Station for PGRB; Fig. 1). A monthly quantile mapping approach (Gudmundsson et al., 2012) with monthly bias correction factors were used for the bias correction of ERA-40 and ERA-Interim.
For PGRB, ERA-Interim data (2008–2018) were bias corrected to Peyto Main Station observations from 2013–2018, and ERA-40 data (1965–1975) were bias corrected to the archived observations from the station for the common overlap period of 1992–2001, as described by Pradhananga et al. (2021). For AGRB, ERA-Interim data were bias corrected to 2014–2018 observations at the Athabasca Moraine Station. There were not any in situ meteorological observations available at AGRB prior to 2014. Therefore, the biases in ERA-40 for AGRB were identified by comparing the overlapping period of ERA-40 and bias-corrected ERA-Interim, similar to the procedure outlined by Krogh and Pomeroy (2018).
2.3 CRHM-glacier model
The CRHM-glacier model (Pradhananga and Pomeroy, 2022), developed in the Cold Regions Hydrological Modelling platform (Pomeroy et al., 2007), was applied in this study to evaluate the impacts of changes in climate and in glacier configuration on the hydrology of glacierized basins. CRHM-glacier is a physically based, flexible, multi-physics hydrological model (Pradhananga and Pomeroy, 2022). It downscales and distributes meteorological variables (shortwave and longwave radiation, air temperature, relative humidity, wind speed, precipitation, and its phase) to differing slopes, aspects, elevations, and groundcover defined by hydrological response units (HRUs), using in-built algorithms and macros (Ellis et al., 2010; Harder and Pomeroy, 2013). The HRUs used to discretize these basins are presented in Pradhananga and Pomeroy (2022). CRHM-glacier simulates the hydrology of both glacier and non-glacier areas in a basin. It redistributes snow by coupling the blowing snow transport and sublimation processes with snow avalanching. The implementation used here does not calculate ice flow. Melt energies for snow and ice melt are calculated separately, based on Snobal and energy budget glacier melt modules, respectively, to calculate snow, firn, and ice mass balances (Pradhananga and Pomeroy, 2022). Meltwater routing is through three glacier reservoirs (snow, firn, and ice), modified to the de Woul et al. (2006) approach. Once water leaves the glacier, rain and meltwater are routed further into the soil surface, subsurface, and groundwater using well-developed alpine routing routines (Fang et al., 2013). The model includes the calculation of actual evapotranspiration, using the Penman–Monteith method and soil moisture and groundwater dynamics, based on infiltration to frozen and unfrozen soils, and the use of saturated and unsaturated hydraulic conductivities to calculate flow velocities in porous media. The model parameters are not calibrated from its fit to streamflow. Parameters are selected primarily from local and regional observations (e.g., temperature and precipitation lapse rates), and its operation has been verified against observed albedo, mass balance, melt rate, and streamflow at PGRB and AGRB (Pradhananga and Pomeroy, 2022).
2.4 Modelling scenarios
CRHM-glacier was run to simulate the hydrological responses of the two glacier research basins to four experimental scenarios. Observed glacier configurations, with “past” corresponding to 1966 (PGRB) and 1983–1984 (AGRB) and “present” to 2014 (PGRB) and 2011–2014 (AGRB), were set as initial conditions for two modelling scenarios in each basin. Initializing the model with observed glacier configurations, and restricting simulation periods to 1 decade, compensated for the lack of ice flow dynamics in the model scenarios.
Glacier configuration maps for the two periods were prepared from available digital elevation model (DEM) and land cover information. These maps were used to delineate the configuration, namely the accumulation (snow- or firn-covered) and ablation (ice-exposed) areas and to determine ice elevations, slopes, and aspects. The glacier area was taken as the sum of the snow-, firn-, and ice-exposed areas. The accumulation area ratio (AAR) of the glaciers was calculated as the ratio of snow-/firn-covered area to the total glacier area.
A topographic map of Peyto Glacier from 1966 (Sedgwick and Henoch, 1975) was used to prepare the past glacier configuration scenario. Both the 1966 DEM (10 m resolution) and the 1966 land cover map were developed from this topographic map, which was produced from aerial photographs taken in August 1966. The 2014 DEM was prepared at 10 m resolution from aerial photographs taken during July and September 2014 by Parks Canada over Banff National Park and made available for this study. The land cover map for the present basin was prepared based upon the Landsat image taken on 18 August 2014. Bolch et al. (2010) found only 1.7 % deviation between aerial photograph and satellite imagery approaches for delineating glacier area and snow/firn/ice coverage. For AGRB, DEM, and land cover map were not available from the 1960s. Therefore, DEM at 20 m horizontal resolution from 1983 (Canadian Digital Elevation Model – CDEM) and the satellite image taken on 28 July 1984 (Landsat 5) was used to prepare the past glacier configuration. The 2011 DEM (20 m resolution from the Japan Aerospace Exploration Agency – JAXA) and the 2014 satellite image (Landsat 8) taken on 18 August were used to prepare the present glacier configuration.
The models were then run for two climate periods, namely past (1965–1975) and present (2008–2018). A novel approach was used in that past and present climate forced both past and present glacier configurations to diagnose how past glaciers would respond to the present-day climate and present-day glaciers to the past climate. Therefore, there was a combination of four model simulation scenarios, A–D, using 2 separate decades of climate forcing from past and present periods, with past and present glacier configurations as follows:
- A.
Past climate – past glacier
- B.
Past climate – present glacier
- C.
Present climate – past glacier
- D.
Present climate – present glacier.
Based on these four experimental scenarios (A–D), five comparisons (C1–C5; Table 1) were employed to diagnose the impacts of climate and glacier changes on streamflow. C1 represents realistic conditions of both climate and glacier configuration; it compares A and D, i.e., past climate – past glacier with present climate – present glacier. The other comparisons are falsified modelling experiments to segregate the impacts of changing climate and glacier configurations. C2 and C5 consider only changes in the glacier configuration, while keeping the climate fixed in either the past or present. C3 and C4 compare the impacts from changing the climate, while keeping the glacier configuration constant as either the past or present glacier. Simulated runoff from these model outputs was examined to diagnose the hydrological response to both glacier change and climate change.
Statistical tests, the Student's t test and the Wilcoxon signed-rank test (Wilcoxon, 1945) in the R environment (R Core Team, 2017), were applied to test the significance of the changes between the results obtained from the model scenarios. These tests were used to assess whether one population of model output metrics is statistically distinguishable from the other. All tests were conducted at the 5 % level of significance.
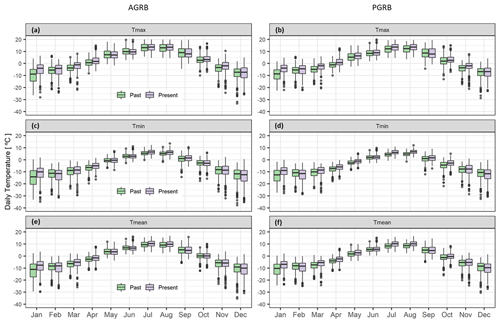
Figure 2Monthly means of daily maximum (a, b), minimum (c, d), and mean (e, f) temperature comparison between two periods, i.e., past (1965–1975) and present (2008–2018). (a, c, e) AGRB. (b, d, f) PGRB.
3.1 Change in climate
Air temperature and precipitation over PGRB and AGRB were analysed for the 2 decades – 1965–1975 (past) and 2008–2018 (present). Daily mean (Tmean), maximum (Tmax), and minimum (Tmin) temperature (Fig. 2 and Tables S2–S4) and monthly precipitation (Fig. 3 and Table S1), averaged and aggregated over the two climatic periods, were compared using C1.
Annual and seasonal average temperatures generally increased in the present decade compared to the past, except for the summer maximum at AGRB. Annual Tmax increased by 1 ∘C at AGRB and by 1.5 ∘C at PGRB. Analysis of the monthly time periods also shows that air temperatures have increased at both glaciers. The greatest increases in the monthly Tmax were in January, when they rose by 5.1 ∘C at AGRB and 4.8 ∘C at PGRB. Monthly Tmin at AGRB increased by 3.6 ∘C in January and by 1 ∘C in July, and that at PGRB increased by 3.5 ∘C in January and 1.9 ∘C in July. Annual Tmean at AGRB increased by 0.5 ∘C, and that at PGRB increased by 1 ∘C. Temperature differences over time that were found to be significantly different from zero are more consistently evident at PGRB than at AGRB.
Annual precipitation increased slightly in the present decade compared to the past (Fig. 3 and Table S1), with 15.2 mm (2.5 %) at AGRB and 71.7 mm (10.3 %) at PGRB. But these differences are not statistically significant. Winter (December–February) precipitation decreased at both basins, by 49.5 mm at AGRB and by 35.2 mm at PGRB. But precipitation in the other seasons (spring, summer, and fall) increased by 13.1, 38.5, and 13.1 mm, respectively, at AGRB and by 29.7, 50.2, and 27.0 mm, respectively, at PGRB. The increases in summer precipitation at both basins, and the decrease in winter precipitation at AGRB, were statistically significant (Table S1).
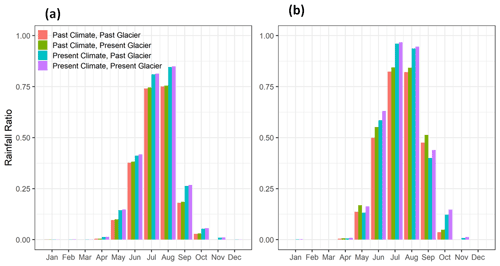
Figure 4Mean monthly rainfall ratios simulated for the four model run scenarios, with A–D combining the past and present climate and glacier. (a) AGRB. (b) PGRB.
There was a statistically significant increase in the rainfall ratio (rainfall divided by total precipitation), for D compared A (C1) in both research basins (Fig. 4), from 0.161 to 0.232 (44 %) at AGRB and from 0.220 to 0.283 (29 %) at PGRB (Table S5). The average annual rainfall ratios increased with reductions in glacier area and surface elevation; at AGRB there was an increase from 0.161 to 0.163 for the past climate (C2) and from 0.163 to 0.232 for the present climate (C5) and at PGRB from 0.220 to 0.283 for the past climate (C2) and from 0.268 to 0.283 for the present climate (C5). However, the increase in rainfall ratios was mainly due to changes in climate (temperature increases) over time. The average annual rainfall ratio for the past glacier configuration (C3) increased from 0.161 to 0.229 at AGRB (statistically significant) and from 0.220 to 0.268 at PGRB, and for the present glacier configuration (C4), the ratio increased from 0.163 to 0.232 at AGRB (statistically significant) and from 0.234 to 0.283 at PGRB.
3.2 Change in glacier configuration
Figure 5 compares the configuration of both glaciers between the two periods and shows changes in the accumulation and ablation areas. During the period 1966–2014, the area of Peyto Glacier shrank from 14.4 to 9.9 km2 (31 %) and its AAR dropped from 0.75 to 0.35, exposing more ice in 2014, which is more than double the area exposed in 1966. The exposed ice area increased from 3.6 to 6.4 km2 (78 %), whereas the snow/firn area decreased from 10.8 to 3.5 km2. Though to a lesser degree than Peyto Glacier, the area of Athabasca Glacier also decreased from 18 to 16.9 km2 (6 %), and its AAR decreased from 0.76 to 0.47. The exposed ice area of Athabasca increased from 4.3 to 9.0 km2 (109 %), and the snow/firn area decreased from 13.6 to 7.9 km2. The firn line moved to a higher elevation in both glaciers, and glacier surfaces have become steeper from the past to present period; the slope of the glacier increased from 14.2 to 16.8 in AGRB and that increased in PGRB from 19.9 to 22.0. The other change in the two glacier configurations was in elevation; the mean glacier surface elevation for Peyto Glacier decreased from 2628 to 2615 m, while that of Athabasca Glacier increased from 2799 to 2826 m.
3.3 Change in glacier mass balance
Seasonal and annual mass balance for AGRB and PGRB from the four model scenarios, A–D, are presented in Fig. 6. The results from the statistical analysis are presented in Table S7. Except for the change in winter mass balance between A and D (C1), the mass balance changes are not statistically significant at AGRB. There were significant changes in winter and annual mass balances between past and present climates and glaciers at PGRB (C1). Mean annual winter accumulation decreased from an average of 586 mm (1965–1975) to 324 mm (2008–2018), resulting in negative mean annual mass balances, from −271 mm in the past climate to −733 mm in the present climate (Table S7). These changes are more due to the change in climate than the change in glacier configuration. Summer ablation increased significantly from past to present climate for both past and present glacier configurations (C3 and C4). The changes are not statistically significant in the model runs for C2 and C5. However, the past glacier configurations resulted in greater winter snow accumulations in both basins for both past and present climates.
3.4 Change in runoff and runoff generation processes
Figure 7 shows runoff and volumetric melt components of runoff from AGRB and PGRB for A and D scenarios (C1). Snowmelt runoff dominated both of the basins in comparison to the rainfall runoff, ice melt runoff, and firn melt runoff. The present climate and present glacier configurations (D) produced more runoff than the past climate and past glacier configurations (A). There was a 19 % significant increase (p = 0.005) in the mean annual runoff, from 1581 to 1888 mm at PGRB (Table S5). This was mainly due to an increased contribution from ice melt, which was from 265 to 667 mm. There was a decrease in the mean annual snowmelt and firn melt, but the changes in these and the other fluxes were not statistically significant. For AGRB, the 3 % increase in runoff from 1320 to 1365 mm was not statistically significant (p = 0.578; Table S5), though there was a significant increase in rainfall from 175 to 262 mm. Monthly statistical values are shown in Table S6. AGRB experienced increased snowmelt and firn melt but decreased ice melt.
Snowmelt-dominated basin runoff provided 52 %–70 % and 52 %–53 % of all runoff for PGRB and AGRB, respectively. At PGRB, rainfall–runoff provided 19 %–20 % of basin runoff, which exceeded ice melt and firn melt contributions, except for ice melt in the current climate and glacier configuration. The sum of firn melt and ice melt contributions at PGRB increased from 27 % in the past to 43 % in the present (C1). At AGRB, rainfall–runoff provided 13 %–19 % of basin runoff and was smaller than either firn melt or ice melt contributions. Firn melt was smaller than both snowmelt and ice melt. The sum of firn melt and ice melt contributions at AGRB decreased slightly from 61 % in the past to 59 % in the present. Basin runoff increased by 19 % at PGRB but only by 3 % at AGRB from the past to present climate for the present glacier configuration (C1). This increase was due to increases in rainfall (statistically significant), snowmelt, and firn melt at AGRB, whereas it was due to an increase in rainfall and a remarkable 152 % increase in ice melt (statistically significant) at PGRB (Table S5). Snowmelt declined 12 % at PGRB. Basin runoff increased due to the warming climate for both past and present glaciers (C3 and C4), whereas it decreased with decreasing glacier configurations for both the past and present climates (C2 and C5).
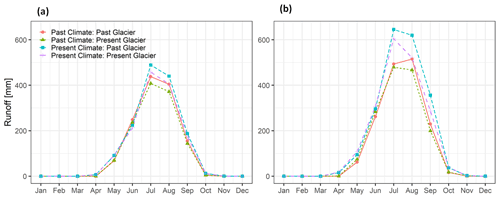
Figure 8Monthly averaged runoff simulated using the past and present glacier and climate scenarios. (a) AGRB. (b) PGRB.
Monthly averaged runoff from the four model scenarios is presented in Fig. 8. The glaciers in AGRB and PGRB produced more runoff in the recent period (D) than in the past (A). Both past and present glaciers produced more runoff in the present than in the past climate. However, the past glaciers generated more streamflow compared to the present glaciers with both present and past climates. As the climate shifted, there was a shift in the peak from August in the past (A) to July in the present (D) at PGRB.
Significant changes in runoff, firn melt, and snowmelt occurred for PGRB, suggesting that the increase in runoff over time (C3 and C4) was due to an increase in firn melt and ice melt (Table S5). The large loss of firn coverage from past to present at PGRB resulted in a decrease of firn melt by 65 % (from 414 to 146 mm), when the past glacier configuration was replaced by the present one, with the climate for both glacier configurations held constant at the present climate (C5). In the case of AGRB, only increases in rainfall in the C3 and C4 and in snowmelt in C4 were significant. More rainfall occurred with both past and present glacier configurations.
The glaciohydrological model, CRHM, was used to simulate the headwater hydrology of two glacierized mountain basins with four scenarios by combining the climate and glacier configurations of two periods, mid-20th century and early 21st century. These scenarios were used to diagnose the hydrological responses to changes in glacier and climate, individually and jointly presenting how glaciers in the past would respond to present-day climate and glaciers today to past climate.
Changes in glacier configurations included changes in glacier extent, glacier surface elevation and slope, and change in AAR, which also changed the albedo feedback of glacier surfaces. Compared to the past, present glacier areas have declined, elevations have decreased/increased, surface slopes have become steeper, and AARs have become smaller, exposing more ice, and thus reduced the glacier albedos.
Air temperatures increased from past to present; the changes were more often significant at PGRB than at AGRB. Though there was not much change in the total precipitation, rainfall ratios increased in the present compared to past due to changes in both climate and glacier configuration, and this caused reduced snowfall at both basins. The differences for the same climate were due to the change in glacier configurations; for instance, the present glacier configurations feature a smaller glacier surface at a lower surface height than past configurations do. Both factors contribute to increased rainfall ratios. Increases in the rainfall ratio in these basins are consistent with other studies, for example, in western Canada by DeBeer et al. (2016) and in Europe by Hynčica and Huth (2019). In the case of glacier mass balance, the present climate and present glacier were responsible for making the winter mass balance less positive. Compared to the present glacier configuration, the past glacier gained more mass during winter.
There was a reduction in peak monthly flows from both basins as the glacier area declined over time with the climate held constant (Fig. 8). However, with the climate changing and the glacier configuration held constant, peak monthly flows increased over time. The combination of moving from the past to present climate and changing glacier configuration shifted peak monthly flows forward by a month at PGRB, but the impact of changing climate was greater than that of the changing glacier configuration on this shift. The shift in PGRB's peak monthly flow from August to July is consistent with the future prediction by Kienzle et al. (2012), for the Cline River watershed, that spring runoff and peak streamflow would shift 18–26 d advance in the 21st century (2020–2080) compared to the baseline period (1961–1989).
In general, there was increased runoff in the present compared with the past for both basins, particularly from PGRB. This shows that these glaciers in Alberta, in the cold, high-elevation headwaters on the northeastern slopes of the Canadian Rockies, are still in the initial phase of warming-induced increased runoff, in contrast to most glaciers in British Columbia, including the more temperate southwestern slopes of the Canadian Rockies (Stahl and Moore, 2006) and western Canada in general (DeBeer et al., 2016). However, this is similar to the results of Chernos et al. (2020), who projected glacier contributions to streamflow to increase till the middle of the 21st century (2040) and to decrease thereafter for the Athabasca River. Moore et al. (2009) also noted increasing trends of runoff in glacierized basins in relatively colder northwest British Columbia and southwest Yukon. Casassa et al. (2009) generalized that high-elevation basins and/or basins with high glacierization were experiencing increasing runoff trends around the world.
The novel model falsification approach of using the past glacier configuration with present climate and the present glacier configuration with past climate provided a means to separate the combined and individual impacts of climate change and glacier recession on the headwater hydrology and water balance of glacierized basins. With fixed glacier configurations (C3 and C4), the impact of climate on glacier hydrology could be analysed, and using the same climate to force past and present glacier configuration models (C2 and C5) provided an explicit separation of the impacts of changes in glacier configuration on glacier hydrology.
The warmer temperatures and increased rainfall ratio in the present climate led to increased glacier runoff from both basins. However, the reduced glacier extent in the present glacier configuration resulted in decreased runoff from both basins, counteracting the direct impacts of climate change on the basins. In summary, the outputs show that changes in both climate and basin configurations were causing changes in the melt rate and runoff. Compared to the past climate and past glacier configuration, the present climate and present glacier configuration provided more runoff in both basins, although there were significant losses of glacier area over the last 5 decades.
This study investigated the influence of snow and glaciers on headwater hydrology in two mountain basins in the Canadian Rockies, where a warming climate and glacier retreat continue to cause concern about changes in high mountain hydrology.
There was an increase in air temperature, mainly in daily maximum and winter minimum temperatures. Total precipitation has not increased, but the rainfall ratio has increased with the shift in climate. Both present climate and present glacier caused an increase in rainfall ratio compared to past climate and past glacier. Decreases in winter precipitation were balanced by increased precipitation in the other seasons, some of which fell as rainfall. Both mass balance studies and analysis of satellite imagery show that the glaciers are losing mass and area, and that the exposure of ice at the glacier surfaces has increased. The fractional glacier retreat is lower at AGRB (6 %) than at PGRB (31 %), whereas the fractional change in the exposure of ice is higher at AGRB (109 %) than at PGRB (78 %). The retreat of the glaciers has led to reductions in glacierized areas and changes in elevation and slope of the glacier surfaces.
The study used a novel approach to apply present climate forcings to drive hydrological modelling, using past glacier configurations, and past climate forcings to drive modelling, using present glacier configuration, so that the impacts of changes in glacier configuration and climate on glacier hydrology could be explicitly separated. The modelling results presented here show that glacier retreat and ablation are due to the joint effect of warming climate and an increase in ice exposure, which increased both seasonal melt and runoff. The sum of firn melt and ice melt contributions to annual discharges increased from the past climate and glacier to the present climate and glacier at PGRB from 27 % to 43 % and decreased slightly at AGRB from 61 % to 59 %. Increased streamflow discharges (3 %–19 %) were due to climate warming and were somewhat limited by glacier retreat and areal decreases. The model results indicated that streamflow from the glaciers increased in the present climate (2008–2018) from the past climate (1965–1975), despite reductions in glacier area and volume. Such a modelling approach is important for diagnosing the hydrological responses from a glacierized basin in the context of climate change and variability. The results suggest that the increased exposure of glacier ice and lower surface elevation due to glacier thinning was less influential than climate warming in increasing streamflow.
CRHM is available at https://research-groups.usask.ca/hydrology/modelling/crhm.php#TechnicalDetails (Centre for Hydrology, 2022).
The dataset for PGRB is publicly available from Federated Research Data Repository at https://doi.org/10.20383/101.0259 (Pradhananga et al., 2020).
The supplement related to this article is available online at: https://doi.org/10.5194/hess-26-2605-2022-supplement.
DP and JWP conceptualized the research. DP did the analysis and prepared the draft. JWP instrumented the research basins, guided the research methods, and edited and revised the paper.
The contact author has declared that neither they nor their co-author has any competing interests.
Any reference to specific products is for informational purposes and does not represent a product endorsement.
Publisher's note: Copernicus Publications remains neutral with regard to jurisdictional claims in published maps and institutional affiliations.
The authors wish to acknowledge the decades of extremely challenging field research at Peyto and Athabasca glacier basins by dozens of scientists and students over the decades. Meriting special mention are D. Scott Munro, of the University of Toronto, and Michael N. Demuth, of Natural Resources Canada, whose legacy of research on Peyto Glacier, including meteorological and mass balance observations, made this study possible. Special mention also goes to the prescient scientists of the International Hydrological Decade, who established Peyto Glacier as a research site, including Gordon Young of Environment Canada and Wilfrid Laurier University. Additional data were provided by Environment and Climate Change Canada's Meteorological Service of Canada and Water Survey of Canada, Parks Canada, and Alberta Environment and Parks. Tom Brown and Xing Fang of the Centre for Hydrology supported CRHM development and operation/parameterization, respectively. Nammy Hang Kirat of The Small Earth Nepal supported satellite image interpretation and GIS maps. Special thanks go to the reviewers (anonymous reviewer no. 1 and Eleanor Bash), for their careful and critical review of the paper and their several insightful comments and constructive suggestions, which helped to improve the paper.
Funding for this study has been provided by the Canada Research Chairs, the Canada Foundation for Innovation, the Natural Sciences and Engineering Research Council of Canada through its Discovery Grants, and the Changing Cold Regions Network and the Canada First Research Excellence Fund's Global Water Futures Programme.
This paper was edited by Carlo De Michele and reviewed by Eleanor Bash and one anonymous referee.
Barry, R. G.: The status of research on glaciers and global glacier recession: a review, Prog. Phys. Geog., 30, 285–306, https://doi.org/10.1191/0309133306pp478ra, 2006.
Bolch, T., Menounos, B., and Wheate, R.: Landsat-based inventory of glaciers in western Canada, 1985–2005, Remote Sens. Environ., 114, 127–137, https://doi.org/10.1016/j.rse.2009.08.015, 2010.
Casassa, G., López, P., Pouyaud, B., and Escobar, F.: Detection of changes in glacial run-off in alpine basins: Examples from North America, the Alps, central Asia and the Andes, Hydrol. Process., 41, 31–41, https://doi.org/10.1002/hyp.7194, 2009.
Castellazzi, P., Burgess, D., Rivera, A., Huang, J., Longuevergne, L., and Demuth, M. N.: Glacial Melt and Potential Impacts on Water Resources in the Canadian Rocky Mountains, Water Resour. Res., 55, 10191–10217, https://doi.org/10.1029/2018WR024295, 2019.
Centre for Hydrology: CRHM: The Cold Regions Hydrological Mode, Centre for Hydrology [code], https://research-groups.usask.ca/hydrology/modelling/crhm.php#TechnicalDetails, last access: 9 May 2022.
Chernos, M., MacDonald, R. J., Nemeth, M. W., and Craig, J. R.: Current and future projections of glacier contribution to streamflow in the upper Athabasca River Basin, Can. Water Resour. J./Rev. Can. des ressources hydriques, 45, 324–344, https://doi.org/10.1080/07011784.2020.1815587, 2020.
Clarke, G. K. C., Jarosch, A. H., Anslow, F. S., Radić, V., and Menounos, B.: Projected deglaciation of western Canada in the twenty-first century, Nat. Geosci., 8, 372–377, https://doi.org/10.1038/ngeo2407, 2015.
Comeau, L. E. L., Pietroniro, A., and Demuth, M. N.: Glacier contribution to the North and South Saskatchewan Rivers, Hydrol. Process., 23, pp. 2640–2653, 2009.
DeBeer, C. M., Wheater, H. S., Carey, S. K., and Chun, K. P.: Recent climatic, cryospheric, and hydrological changes over the interior of western Canada: a review and synthesis, Hydrol. Earth Syst. Sci., 20, 1573–1598, https://doi.org/10.5194/hess-20-1573-2016, 2016.
Dee, D. P., Uppala, S. M., Simmons, A. J., Berrisford, P., Poli, P., Kobayashi, S., Andrae, U., Balmaseda, M. A., Balsamo, G., Bauer, P., Bechtold, P., Beljaars, A. C. M., van de Berg, L., Bidlot, J., Bormann, N., Delsol, C., Dragani, R., Fuentes, M., Geer, A. J., Haimberger, L., Healy, S. B., Hersbach, H., Hólm, E. V., Isaksen, L., Kållberg, P., Köhler, M., Matricardi, M., Mcnally, A. P., Monge-Sanz, B. M., Morcrette, J. J., Park, B. K., Peubey, C., de Rosnay, P., Tavolato, C., Thépaut, J. N., and Vitart, F.: The ERA-Interim reanalysis: Configuration and performance of the data assimilation system, Q. J. Roy. Meteorol. Soc., 137, 553–597, https://doi.org/10.1002/qj.828, 2011.
Demuth, M. N. and Keller, R.: An assessment of the mass balance of Peyto glacier (1966-1995) and its relation to Recent and past-century climatic variability, in: Peyto Glacier: One Century of Science, edited by: Demuth, M. N., Munro, D. S., and Young, G. J., National Hydrology Research Institute, Saskatoon, Saskatchewan, pp. 83–132, 2006.
de Woul, M., Hock, R., Braun, M., Thorsteinsson, T., Jóhannesson, T., and Halldórsdóttir, S.: Firn layer impact on glacial runoff: a case study at Hofsjökull, Iceland, Hydrol. Process., 20, 2171–2185, https://doi.org/10.1002/hyp.6201, 2006.
Ellis, C. R., Pomeroy, J. W., Brown, T., and MacDonald, J.: Simulation of snow accumulation and melt in needleleaf forest environments, Hydrol. Earth Syst. Sci., 14, 925–940, https://doi.org/10.5194/hess-14-925-2010, 2010.
Fang, X. and Pomeroy, J. W.: Diagnosis of future changes in hydrology for a Canadian Rockies headwater basin, Hydrol. Earth Syst. Sci., 24, 2731–2754, https://doi.org/10.5194/hess-24-2731-2020, 2020.
Fang, X., Pomeroy, J. W., Ellis, C. R., MacDonald, M. K., DeBeer, C. M., and Brown, T.: Multi-variable evaluation of hydrological model predictions for a headwater basin in the Canadian Rocky Mountains, Hydrol. Earth Syst. Sci., 17, 1635–1659, https://doi.org/10.5194/hess-17-1635-2013, 2013.
Fountain, A. G. and Tangborn, W. V.: The effect of glaciers on streamflow variations, Water Resour. Res., 21, 579–586, https://doi.org/10.1029/WR021i004p00579, 1985.
Gudmundsson, L., Bremnes, J. B., Haugen, J. E., and Engen-Skaugen, T.: Technical Note: Downscaling RCM precipitation to the station scale using statistical transformations – a comparison of methods, Hydrol. Earth Syst. Sci., 16, 3383–3390, https://doi.org/10.5194/hess-16-3383-2012, 2012.
Harder, P. and Pomeroy, J. W.: Estimating precipitation phase using a psychrometric energy balance method, Hydrol. Process., 27, 1901–1914, https://doi.org/10.1002/hyp.9799, 2013.
Harder, P., Pomeroy, J. W., and Westbrook, C. J.: Hydrological resilience of a Canadian Rockies headwaters basin subject to changing climate, extreme weather, and forest management, Hydrol. Process., 29, 3905–3924, https://doi.org/10.1002/hyp.10596, 2015.
Hopkinson, C. and Young, G. J.: The effect of glacier wastage on the flow of the Bow River at Banff, Alberta, 1951–1993, Hydrol. Process., 12, 1745–1762, https://doi.org/10.1002/(SICI)1099-1085(199808/09)12:10/11<1745::AID-HYP692>3.0.CO;2-S, 1998.
Hynčica, M. and Huth, R.: Long-term changes in precipitation phase in Europe in cold half year, Atmos. Res., 227, 79–88, https://doi.org/10.1016/j.atmosres.2019.04.032, 2019.
Intsiful, A. and Ambinakudige, S.: Glacier Cover Change Assessment of the Columbia Icefield in the Canadian Rocky Mountains, Canada (1985–2018), Geosciences, 11, 19, https://doi.org/10.3390/GEOSCIENCES11010019, 2021.
Kehrl, L. M., Hawley, R. L., Osterberg, E. C., Winski, D. A., and Lee, A. P.: Volume loss from lower Peyto Glacier, Alberta, Canada, between 1966 and 2010, J. Glaciol., 60, 51–56, https://doi.org/10.3189/2014JoG13J039, 2014.
Kienzle, S. W., Nemeth, M. W., Byrne, J. M., and Macdonald, R. J.: Simulating the hydrological impacts of climate change in the upper North Saskatchewan River basin, Alberta, Canada, J. Hydrol., 412–413, 76–89, https://doi.org/10.1016/j.jhydrol.2011.01.058, 2012.
Krogh, S. A. and Pomeroy, J. W.: Recent changes to the hydrological cycle of an Arctic basin at the tundra–taiga transition, Hydrol. Earth Syst. Sci., 22, 3993–4014, https://doi.org/10.5194/hess-22-3993-2018, 2018.
Marks, D., Winstral, A., Flerchinger, G., Reba, M., Pomeroy, J., Link, T., and Elder, K.: Comparing Simulated and Measured Sensible and Latent Heat Fluxes over Snow under a Pine Canopy to Improve an Energy Balance Snowmelt Model, J. Hydrometeorol., 9, 1506–1522, https://doi.org/10.1175/2008JHM874.1, 2008.
Marshall, S. J., White, E. C., Demuth, M. N., Bolch, T., Wheate, R., Menounos, B., Beedle, M. J., and Shea, J. M.: Glacier Water Resources on the Eastern Slopes of the Canadian Rocky Mountains, Can. Water Resour. J., 36, 109–134, https://doi.org/10.4296/cwrj3602823, 2011.
Moore, R. D., Fleming, S. W., Menounos, B., Wheate, R., Fountain, A., Stahl, K., Holm, K., and Jakob, M.: Glacier change in western North America: influences on hydrology, geomorphic hazards and water quality, Hydrol. Process., 23, 42–61, https://doi.org/10.1002/hyp.7162, 2009.
Neupane, R. P., Adamowski, J. F., White, J. D., and Kumar, S.: Future streamflow simulation in a snow-dominated Rocky Mountain headwater catchment, Hydrol. Res., 49, 1172–1190, https://doi.org/10.2166/NH.2017.024, 2018.
Pomeroy, J. W., Gray, D. M., Brown, T., Hedstrom, N. R., Quinton, W. L., Granger, R. J., and Carey, S. K.: The cold regions hydrological model: a platform for basing process representation and model structure on physical evidence, Hydrol. Process., 21, 2650–2667, https://doi.org/10.1002/hyp.6787, 2007.
Pradhananga, D. and Pomeroy, J. W.: Diagnosing changes in glacier hydrology from physical principles using a hydrological model with snow redistribution, sublimation, firnification and energy balance ablation algorithms, J. Hydrol., 608, https://doi.org/10.1016/j.jhydrol.2022.127545, 2022.
Pradhananga, D., Pomeroy, J., Aubry-Wake, C., Munro, D., Shea, J., Demuth, M., Kirat, N., Menounos, B., and Mukherjee, K.: Hydrometeorological, glaciological and geospatial research data from the Peyto Glacier Research Basin in the Canadian Rockies, Federated Research Data Repository [data set], https://doi.org/10.20383/101.0259, 2020.
Pradhananga, D., Pomeroy, J. W., Aubry-Wake, C., Munro, D. S., Shea, J., Demuth, M. N., Kirat, N. H., Menounos, B., and Mukherjee, K.: Hydrometeorological, glaciological and geospatial research data from the Peyto Glacier Research Basin in the Canadian Rockies, Earth Syst. Sci. Data, 13, 2875–2894, https://doi.org/10.5194/essd-13-2875-2021, 2021.
R Core Team: R: A language and environment for statistical computing, R Foundation for Statistical Computing, Vienna, Australia, https://www.r-project.org/ (last access: 9 May 2022), 2017.
Reynolds, J. R. and Young, G. J.: Changes in areal extent, elevation and volume of Athabasca Glacier, Alberta, Canada, as estimated from a series of maps produced between 1919 and 1979, Ann. Glaciol., 24, 60–65, 1997.
Riedel, J. L., Wilson, S., Baccus, W., Larrabee, M., Fudge, T. J., Fountain, A., and Riedel, C. J. L.: Glacier status and contribution to streamflow in the Olympic Mountains, Washington, USA, J. Glaciol., 61, 8–16, https://doi.org/10.3189/2015JoG14J138, 2015.
Schiefer, E., Menounos, B., and Wheate, R.: Recent volume loss of British Columbian glaciers, Canada, Geophys. Res. Lett., 34, 1–6, https://doi.org/10.1029/2007GL030780, 2007.
Sedgwick, J. K. and Henoch, W. E. S.: 1966 Peyto Glacier Map, Banff National Park, Alberta, Environment Canada, IWD 1010, ., 1975.
Stahl, K. and Moore, R. D.: Influence of watershed glacier coverage on summer streamflow in British Columbia, Canada, Water Resour. Res., 42, 1–5, https://doi.org/10.1029/2006WR005022, 2006.
Tennant, C. and Menounos, B.: Glacier change of the Columbia Icefield, Canadian Rocky Mountains, 1919–2009, J. Glaciol., 59, 671–686, https://doi.org/10.3189/2013JoG12J135, 2013.
Tennant, C., Menounos, B., Wheate, R., and Clague, J. J.: Area change of glaciers in the Canadian Rocky Mountains, 1919 to 2006, The Cryosphere, 6, 1541–1552, https://doi.org/10.5194/tc-6-1541-2012, 2012.
Uppala, S. M., KÅllberg, P. W., Simmons, A. J., Andrae, U., Bechtold, V. D. C., Fiorino, M., Gibson, J. K., Haseler, J., Hernandez, A., Kelly, G. A., Li, X., Onogi, K., Saarinen, S., Sokka, N., Allan, R. P., Andersson, E., Arpe, K., Balmaseda, M. A., Beljaars, A. C. M., Berg, L. Van De, Bidlot, J., Bormann, N., Caires, S., Chevallier, F., Dethof, A., Dragosavac, M., Fisher, M., Fuentes, M., Hagemann, S., Hólm, E., Hoskins, B. J., Isaksen, L., Janssen, P. A. E. M., Jenne, R., Mcnally, A. P., Mahfouf, J.-F., Morcrette, J.-J., Rayner, N. A., Saunders, R. W., Simon, P., Sterl, A., Trenberth, K. E., Untch, A., Vasiljevic, D., Viterbo, P., and Woollen, J.: The ERA-40 re-analysis, Q. J. Roy. Meteorol. Soc., 131, 2961–3012, https://doi.org/10.1256/qj.04.176, 2005.
Wilcoxon, F.: Individual Comparisons by Ranking Methods, Biometrics Bull., 1, 80, https://doi.org/10.2307/3001968, 1945.