the Creative Commons Attribution 4.0 License.
the Creative Commons Attribution 4.0 License.
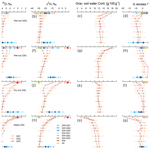
Investigating unproductive water losses from irrigated agricultural crops in the humid tropics through analyses of stable isotopes of water
Amani Mahindawansha
Christoph Külls
Philipp Kraft
Lutz Breuer
Reliable information on water flow dynamics and water losses via irrigation on irrigated agricultural fields is important to improve water management strategies. We investigated the effect of season (wet season and dry season), irrigation management (flooded and non-flooded), and crop diversification (wet rice, dry rice, and maize) on soil water flow dynamics and water losses via evaporation during plant growth. Soil water was extracted and analysed for the stable isotopes of water (δ2H and δ18O). The fraction of evaporation losses were determined using the Craig–Gordon equation. For dry rice and maize, water in shallow soil layers (0 to 0.2 m) was more isotopically enriched than in deeper soil layers (below 0.2 m). This effect was less pronounced for wet rice but still evident for the average values at both soil depths and seasons. Soil water losses due to evaporation decreased from 40 % at the beginning to 25 % towards the end of the dry season. The soil in maize fields showed stronger evaporation enrichment than in rice during that time. A greater water loss was encountered during the wet season, with 80 % at the beginning of the season and 60 % at its end. The isotopic enrichment of ponding surface water due to evaporation was reflected in the shallow soils of wet rice. It decreased towards the end of both growing seasons during the wet and the dry season. We finally discuss the most relevant soil water flow mechanisms, which we identified in our study to be those of matrix flow, preferential flow through desiccation cracks, and evaporation. Isotope data supported the fact that unproductive water losses via evaporation can be reduced by introducing dry seasonal crops to the crop rotation system.
- Article
(2112 KB) - Full-text XML
-
Supplement
(175 KB) - BibTeX
- EndNote
Soil water studies are essential for a better understanding of the role soils play in the hydrological cycle, and to estimate the water budget and water availability for plants, groundwater recharge, other organisms, and solute transport. Stable isotopes of water (δ2H and δ18O) as natural tracers have become a powerful tool for such studies (Kendall and Caldwell, 1999) They are particularly helpful to better understand the evaporation dynamics in soil water (Braud et al., 2009; Kool et al., 2014; Rothfuss et al., 2015) because the composition and distribution of stable isotopes of water in a soil profile provide insight into evaporation fractionation and water flux processes (Wenninger et al., 2010).
The determination of soil evaporation and the fraction of evaporation in relation to total evapotranspiration have been widely studied using several methods for different crops. For example, Liu et al. (2002) studied evapotranspiration from winter wheat and maize, using weighing lysimeters. Zhou et al. (2016) partitioned evaporation and transpiration fluxes for corn, soya bean, grassland, and forests using flux tower measurements. Kool et al. (2014) applied different methods such as chamber measurements, micro-lysimeter, and soil heat pulse to estimate the evaporation. They used stable isotopes of water to separate evaporation from transpiration. Soil isotopic profiles can be subdivided into two parts (Barnes and Allison, 1984); first, the upper part in shallow soil, in which water moves by vapour diffusion and which is affected by evaporation, and second, the lower part in deep soil, where direct flows take place and which is barely affected by diffusion. However, the isotopic composition of soil water is not only affected directly by evaporation, mixing of new and old water (Gazis and Feng, 2004), and altering input signals (Barnes and Turner, 1998), for example through infiltration of rainwater, but also indirectly by other processes such as transpiration (Barnes and Allison, 1988), water transport (Kutilek and Nielsen, 1994; Melayah et al., 1996), and hydrodynamic dispersion (Wang et al., 2017). Transpiration can selectively remove soil water with a specific recharge period and isotopic composition. During dry periods, the isotopic enrichment of shallow soil water is generally driven by evaporation (Gangi et al., 2015; Liu et al., 2015) and is affected by equilibrium and kinetic fractionation (Gat, 1996; Gonfiantini, 1986). Due to this complexity, many experiments on the effects of evaporation on soil water using isotope methods are often restricted to the laboratory-scale or short-term field studies or one particular location (Beyer et al., 2016; Gaj et al., 2016; Oerter and Bowen, 2017; Rothfuss et al., 2015; Sprenger et al., 2017; Twining et al., 2006; Volkmann et al., 2016).
Studying water fluxes in rice-based cropping systems is essential because rice (Oryza sativa L.) is the dominant staple food for nearly half of the world's population; yet, water resources are limited. More than 80 % of the global rice production area is located in Asia (Kudo et al., 2014). Rice is one of the highest-water-consuming grain crops (Janssen and Lennartz, 2007; Mekonnen and Hoekstra, 2011), consuming approximately 30 % of all freshwater resources worldwide (Maclean et al., 2002). Since rice is susceptible to water shortages (Bouman and Tuong, 2001), 80 % of the rice in Asia is cultivated under conventional flooded conditions (Towprayoon et al., 2005); it is also called wet rice, anaerobic rice, or lowland rice. Water scarcity is a severe environmental problem, especially in the irrigation of agricultural land (Pfister et al., 2011). Therefore, water-saving strategies need to be developed to secure rice production (Belder et al., 2004). Introducing non-flooded crops during the dry season (e.g. maize or non-flooded rice, also called dry rice, and aerobic rice or upland rice) is an interesting alternative and has been increasingly applied in food and fodder production in Southeast Asia (FAO, 2016; Timsina et al., 2010). To establish efficient water-saving management based on crop rotation and season, a functional understanding of hydrological processes of these new rice-based cropping systems is required (Daly et al., 2004; Heinz et al., 2013; Zwart and Bastiaanssen, 2004).
Understanding water flow dynamics and unproductive water losses from irrigated soils is still incomplete, particularly for rice-based cropping systems. Unproductive water losses are those that, unlike transpiration, do not lead directly to biomass production and include, for example, leaching, evaporation from the soil, or ponding water (Bouman, 2007). Studies on the effects of evaporation, its seasonal variability, and the impact of various crop rotations are still missing. None of the studies conducted so far have quantified the fraction of evaporation losses in rice-based cropping systems, taking into account the effect of crop species and various growing stages. The objectives of this study are, therefore, (i) to investigate soil water isotopic profiles in order to study the impact of crop species (wet rice, dry rice, and maize) and growing stages on evaporation during the wet and dry season; (ii) to understand flow mechanisms of soil water in the soil matrix of agricultural soils; and (iii) to quantify the fraction of evaporation losses from agricultural fields based on stable isotopes of water.
2.1 Site description and experimental design
The field trial was established at the experimental station of the International Rice Research Institute (IRRI), in Los Baños, Laguna, Philippines (14∘11′ N, 121∘15′ E; 21 m a.s.l.). The experiment was conducted during the wet season (WS) of 2015 and the dry season (DS) of 2016. The average total precipitation during this period was 1700±50 mm during the wet season (WS – June to November) and 300±25 mm during the dry season (DS – December to May). The mean seasonal temperature and relative humidity were 28.5±0.9 ∘C and 83±6 % during the WS 2015, and 27.6±1.8 ∘C and 74±11 % during the DS 2016, respectively. Climate data were obtained from the climate unit at IRRI. Both seasons represented typical weather conditions in the region. The soil type in the study area is classified as a Hydragric Anthrosol (He et al., 2015) with clay-dominated soil texture (Table 1). The clay fraction mainly consists of vermiculite and smectite as three-layer clays and kaolinite as a two-layer clay. Three-layer vermiculite is primarily responsible for the swelling and shrinking of the soil matrix (Tertre et al., 2018).
Table 1Soil texture and average bulk densities of different depths along the soil profile. NA: not available.
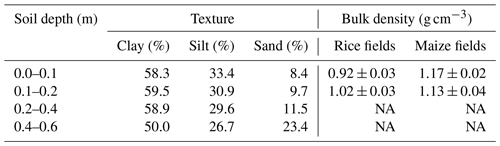
The experimental design (Fig. 1) consisted of nine fields (three wet rice–wet rice, three wet rice–dry rice, and three wet rice–maize) with an average field size of about 540 m2, which are each split into three plots with different treatments. Of these plots, only those with straw application and the control plots (without straw) were used for our experiment. Straw was not applied as a typical mulch layer to reduce evaporation but was partly worked into the soil to reduce crack formation during dry soil conditions to minimize preferential flow losses. During the WS, all nine fields were cropped with wet rice (cultivar NSIC Rc222). During the DS, three fields each were cultivated with wet rice, dry rice (cultivar NSIC Rc192), and maize (Pioneer P3482YR). Wet rice fields were maintained at water-flooded conditions, except for the first and last two weeks between transplanting and harvest (Fig. 2). Dry rice and maize fields were only irrigated when weather conditions suggested a water shortage (i.e. 5–10 times during the growing season for maize fields). Field workers from the IRRI were responsible for watering the dry crops in times of soil water shortages. The decision to water was not set by specific thresholds or indicators but by expert knowledge. The total irrigation amount for wet rice fields was 470±50 mm during the WS and 1270±300, 517±50, and 212±50 mm for wet rice, dry rice, and maize during the DS, respectively. Note that irrigation water was taken from an open reservoir located next to the fields. The reservoir is regularly filled with groundwater that is characterized by a uniform seasonal composition with an isotopically depleted characteristic (Mahindawansha et al., 2018a). Transplanting and harvesting dates for rice were 21 July and 30 October during the WS. During the DS, the transplanting date was 8 January and harvesting dates were 10 April for wet rice, 17 April for dry rice, and 6 January and 11 May for maize in 2016, respectively (Fig. 2).
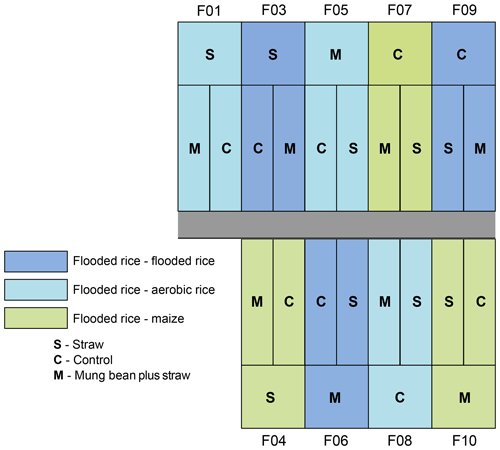
Figure 1Experimental field design. The experiment consisted of nine fields (F) with three different crop rotations and water management practices. During the wet season, all fields were cultivated with wet rice, while during the dry season three fields each were cultivated with flooded rice (wet rice), aerobic rice (dry rice), and maize. Each field is divided into three different treatments (S – straw incorporated in the soil; C – control; and M – straw plus mung bean as an intercrop in the dry to wet transition period). Note that the mung bean plots are not part of this study but are depicted for completeness of the field trial.
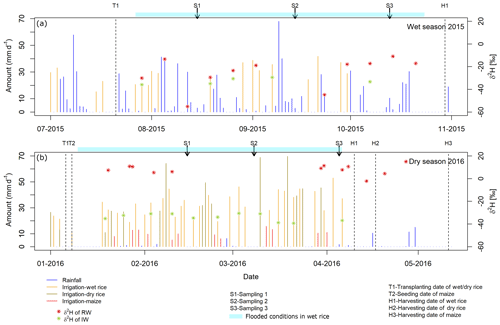
Figure 2Temporal variation of water inputs (rainfall and irrigation water) of wet rice, dry rice, and maize fields for the wet season 2015 (a) and dry season 2016 (b). Three main sampling dates during each season, together with transplanting and harvesting dates, are marked. Values of δ2H are presented for rainwater (RW) and irrigation water (IW) during both seasons.
2.2 Soil and root sampling
Samples were collected during the three main growing stages (GSs) described by Counce et al. (2000), i.e. at the vegetative stage (GS1 – from germination to panicle initiation), the reproductive stage (GS2 – from panicle initiation to flowering), and the ripening stage (GS3 – from flowering to maturity). The growing stages were used as reference points during the growing season (Fig. 2). The growing stages for rice and maize were assumed to be similar to maintain consistency for sampling conditions. Samples were taken on 1 d during each growing stage, namely 26, 55, and 85 d after transplanting during the WS and 40, 60, and 90 d after transplanting during the DS, respectively. Soil cores were taken using a manual soil corer (length – 0.6 m; diameter – 0.05 m). Each core was divided into nine depth intervals from the surface to 0.6 m (0, 0.05, 0.1, 0.15, 0.2, 0.2–0.3, 0.3–0.4, 0.4–0.5, and 0.5–0.6 m). Altogether, 972 samples were taken (9 fields ×2 treatments ×2 seasons ×3 growing stages ×9 soil depths). A plastic ring (diameter – 0.5 m) was used to drain the water around the sampler prior to coring in wet rice fields. Samples were stored in sealed aluminium bags (CB400-420BRZ; 80 mm ×110 mm; Weber Packaging GmbH, Güglingen, Germany) and immediately placed in an ice-filled styrofoam box for transfer to the laboratory where they were kept frozen.
Soil water was extracted from soil aliquots (10–15 g of the sample) via cryogenic vacuum extraction (Orlowski et al., 2013) at the Institute for Landscape Ecology and Resources Management (Justus Liebig University Giessen, Germany) for 4 h at 200 ∘C under a pressure of 0.3 Pa. The gravimetric soil water content along the soil profiles was determined based on the soil weight loss following cryogenic water extraction. Soil water content determined in this way deviates from the classic oven-drying method and results in slightly lower values. In the case of oven drying, samples are taken via stainless-steel cores. These soil cores still have intact pore systems that contain pore water. However, as soil samples taken for cryogenic extraction are disturbed soil samples, they do not include all the pore water. We use the gravimetric soil water content from cryogenic extraction not as an absolute value but rather as a relative value to identify differences along the soil profile. Groundwater and ponded surface water of flooded rice were collected once a week from each plot at existing sampling stations (Heinz et al., 2013). Low-cost car wiper pumps (article no. 103 158; TOPRAN, Ganderkesee, Germany) were used to pump water, with a pumping rate of 40 L h−1, to the sampling container which was installed in the centre of the nine fields (Fig. 1). The installation length below ground was 2.0 m. The groundwater table varied, depending on the season. During the WS the groundwater table was at 0.5–0.6 m below ground and at 0.6–1.7 m during the DS. Rainwater and irrigation water were sampled based on events. For detailed information on the experimental design and sample collection and field preparation, such as puddling and ploughing, see Mahindawansha et al. (2018a, b).
2.3 Isotopic measurements
The oxygen and hydrogen isotopic compositions of the water samples (extracted soil water and liquid samples) were measured via off-axis integrated cavity output spectroscopy (OA-ICOS, DLT-100 Liquid Water Isotope Analyzer; Los Gatos Research Inc., Mountain View, California, USA) and reported in per mil (‰). The analytical precision for δ18O and δ2H was 0.2 ‰ and 0.6 ‰, respectively. All water sources (isotopic data) were checked for spectral interferences using the Spectral Contamination Identifier (LWIA-SCI; Los Gatos Research Inc., Mountain View, California, USA) post-processing software. According to this test, none of the soil water samples were contaminated. The global meteoric water line (GMWL) was defined following Rozanski et al. (1993; ). The local meteoric water line (LMWL) was calculated with , using stable isotope compositions of local precipitation collected from 2000 to 2015 (GNIP-IAEA, 2016). Line-conditioned excess (lc excess) was calculated for soil water samples, as suggested by Landwehr and Coplen (2006), with lc excess = δ2H-aδ18O-b, where a and b refer to the slope and intercept of the LMWL, respectively. The lc excess is an indicator for evaporation, with lower values reflecting larger evaporative losses. We used the lc excess to infer the seasonal dynamics of evaporation fractionation (Sprenger et al., 2017).
2.4 Calculation fraction of evaporation
The joint effect of equilibrium and kinetic isotopic fractionation during the phase transition from liquid water to vapour can be estimated using the Craig–Gordon model (Craig and Gordon, 1965). Sprenger et al. (2017) have recently used Eq. (1) to estimate evaporation from the topsoil (0–0.1 m). Equation (1) is based on the Craig–Gordon model and formulations introduced by Gonfiantini (1986) to estimate the fraction of evaporation loss (FE) from the soil water based on an isotope mass balance approach as follows:
where δS is defined as the isotopic signal of the soil (‰), δp is the original isotopic signal of soil water (‰), δ* is the limiting isotopic enrichment factor (‰), and m is the temporal enrichment slope [–]. In our study, the original isotopic signal δp is the signal of the water input via precipitation or irrigation. During the WS, δp was estimated as the weighted average of the isotopic signals from the frequently occurring large precipitation (larger than 10 mm) events (resulting values are −36.6 ‰ for δ2H and −5.8 ‰ for δ18O). For the DS, we used the weighted mean of the irrigation water as the input signal. We assumed steady-state conditions, as the samples were taken between 10:00 and 12:00. and thus at a time when steady-state conditions in rice fields can be assumed (Wei et al., 2015). Variables δ* and m were calculated following Eqs. (2) and (3), respectively, as described in Benettin et al. (2018) and Gibson et al. (2016):
where δA is the isotopic composition of atmospheric vapour (‰) (assuming that the isotopic composition of atmospheric vapour is in equilibrium with precipitation; see also Eq. (4) in the Supplement), RH is the relative humidity, εk is the kinetic fractionation factor (‰), and α+ [–] and ε+ (‰) are equilibrium fractionation factors. The temperature-dependent parameter α+ was calculated separately for δ2H and δ18O (Benettin et al., 2018). Furthermore, εk was calculated according to Benettin et al. (2018), presuming diffusive transport in soil pore spaces (Barnes and Allison, 1983). The equilibrium isotopic separation between liquid and vapour was computed as (‰) (Benettin et al., 2018). As part of the calculation of εk, the aerodynamic diffusion parameter n [–] has to be set. It reaches 1 when the soil is dried to residual moisture levels (Mathieu and Bariac, 1996) and presents turbulent conditions. We anticipated n=0.5 for wet rice fields with saturated soils (Good et al., 2014), n=0.7 for dry rice, and n=0.9 for maize.
2.5 Statistical analysis
We tested for significant statistical differences (p≤0.05) of stable isotopes of water (δ2H and δ18O) during seasons, growing stages, and treatments between all water sources. Normal distribution was tested with the Shapiro–Wilk test and homogeneity of variances with the Fligner-Killeen test (Python 2.7.10.0). Because of the non-normal distribution of data, we further carried out a non-parametric rank-based test that considered no ties. The isotopic values of the two treatments with straw and without straw as a control plot were combined for each crop for further analysis, as there were no significant differences for stable isotopes of water between the two treatments (p>0.05).
3.1 Soil water isotopic distribution
The original isotopic signal of the incoming water changed depending on the season, especially during the WS. As a result of frequent precipitation events with different amounts introducing strong variations in the isotopic composition (δ2H from −55.20 ‰ to −10.89 ‰ and δ18O from −7.91 to −2.54 ‰), the isotopic signal of the incoming water varied significantly (Fig. 2). Both δ2H and δ18O values of ponded surface water and groundwater were higher at the beginning of each season and decreased towards the end (Fig. 3). During both seasons, ponded surface water and groundwater under wet rice showed a relatively similar range of isotopic composition with no statistically significant differences (Table 2). A distinct difference in the composition of groundwater (GW) was only observed under maize in the DS. Stable isotope compositions of irrigation water were not significantly different between seasons. Rainwater was isotopically similar to groundwater and ponded surface water during the WS unlike during the DS, where it was significantly different.
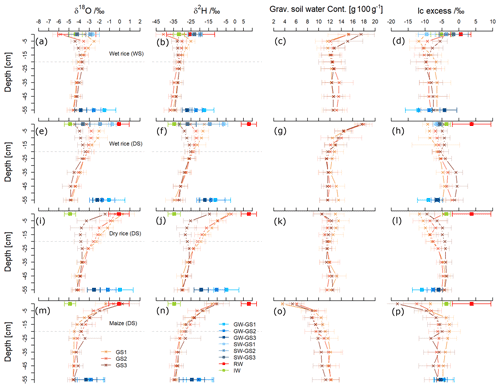
Figure 3Depth profiles of means ± standard deviation for δ18O/‰, δ2H/‰, gravimetric soil water content (gram per 100 g), and lc excess/‰ from three main growing stages (GS1 to GS3) of wet rice (a–d) during the wet season (WS) and wet rice (e–h), dry rice (i–l), and maize (m–p) during the dry season (DS). Seasonal averages ± standard deviation of all water sources: rainwater (RW), irrigation water (IW), groundwater (GW), and ponded surface water (SW). Isotopic values are displayed at the top and bottom of the soil profiles.
Table 2Mean ± standard deviation (SD) of all water samples (rainwater weighted mean – RW; irrigation water – IW; groundwater – GW; and ponded surface water – SW) from different crops (wet rice, dry rice, and maize) during the wet season (WS) and dry season (DS).
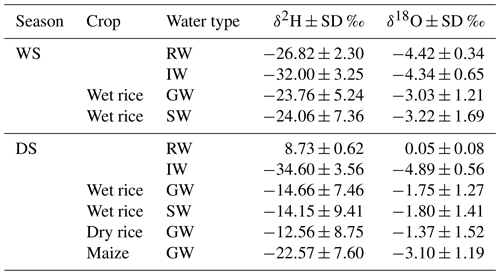
Figure 3 displays the δ2H and δ18O together with the water content and lc excess values in soil water as a function of soil depth during GS1, GS2, and GS3 of wet rice during the WS, along with wet rice, dry rice, and maize during the DS with the standard deviation of the replicates. The range of isotopic composition of rainwater and irrigation water defines the water input to the system for each season (average values are presented in Table 2). The isotopic composition of soil water from fields with different crops during the DS was statistically different (more enriched) from the wet rice during the WS. Within the DS itself, there was a tendency for more depleted conditions to occur in the upper soil horizons of wet rice compared to maize and dry rice. We did not find such a distinct difference for the soil layers below 0.2 m. Results for GS2 and GS3 of maize and wet rice were statistically different during the DS, and maize and dry rice were statistically different, except for the GS3 of dry rice. The isotopic signals of the soil profiles down to a depth of ∼0.2 m were highly variable and became more stable further below. Therefore, soil water isotopic values can be divided into two categories, namely shallow soil water, from 0 to 0.2 m, and deep soil water, from 0.2 to 0.6 m. In the wet rice soil, the isotopic values increased to a depth of 0.05 m and then decreased again until about 0.2 m (Fig. 3a, b, e, and f). Interestingly, in wet rice soils, the depth of the highest isotope enrichment, which is just below the soil surface, decreased in deeper soil layers during the growing period from GS1 to GS3 in both seasons. In contrast, the shape of the isotopic profiles of dry rice and maize follow a different pattern compared to wet rice, with higher δ2H and δ18O values at the soil surface and an exponential decrease down to around 0.2 m soil depth (Fig. 3i, j, m, and n). The isotopic composition of shallow soil in dry rice fields decreased from GS1 to GS3, where the values were stable in maize fields during all phases of plant growth. The isotopic values in deep soil were nearly stable in all the profiles, regardless of the crop, during both seasons.
Maize was characterized by dry soil conditions at the surface and shallow depths compared to both rice varieties (Fig. 3c, g, k, and o). The highest water content was found for wet rice at the surface soil (17.7±1.2 %), and it was nearly constant below a depth of 0.2 m (12.0±1.3 %) during both seasons. The water content in dry rice soils was rather evenly distributed along the soil profile, except for the soil surface. Soils under maize were getting drier as plants were growing, while such clear patterns were not observed for the rice crops.
We found an exponential increase in the lc excess along the soil profile, particularly for maize but also, though less apparent, for dry rice soils (Fig. 3l and p). For wet rice during the DS, the exponential pattern was even less obvious, but shallow soil layers still depicted lower lc excess from −10 ‰ to −5 ‰ than in deeper soil layers, with values from −5 ‰ to 0 ‰. In contrast, lc excess values of shallow soils in wet rice fields of the WS (Fig. 3d) generally decreased with depths of up to 20 cm and then levelled out at around −7 ‰ to −9 ‰. These patterns indicate a higher evaporation signal in shallow soils for the DS crops compared to the WS crop. The highest evaporative fractionation was found near the surface in maize fields with significantly lower lc excess values during the last growing stage (GS3). For maize, the lc excess values decreased in most soil layers from GS1 to GS3, which was the opposite for dry and wet rice during the DS. No distinct, clear pattern could be found along the growing stages for wet rice during the WS. The lc excess for rainwater for both seasons was mostly positive and ranged from 0 ‰ to 9 ‰ during the DS and from −2 ‰ to 4 ‰ during the WS. The positive lc excess for rainfall values during the DS indicates the influence of changes in moisture sources of the rainfall. Irrigation water has a nearly similar mean of −5 ‰ for the WS and −4 ‰ for the DS.
The δ2H and δ18O values of soil water plot on a line below the LMWL due to the evaporation effect (Fig. 4). The slope of the regression line and coefficient of determination (R2) were higher in the DS (avg slope =5.1; R2=0.92) than during the WS (avg slope =3.5; R2=0.54). Soil water δ2H and δ18O compositions were higher (enriched) in shallow soils and deviated more strongly from the LMWL than soil water from deep soils. We observed lower slopes and more clustered data points in wet rice soil during the WS, indicating lower soil evaporation compared to the DS. During the WS, several shallow soil isotopic values plotted close to the LMWL, and some deep soil values deviated more from the LMWL (Fig. 4a–c). For wet rice during WS, the isotopic composition of soil water is more depleted than the weighted isotopic composition of rainfall. This bias is caused by selective infiltration of large events and has been accounted for by using the weighted average isotopic composition of rainfall events larger than 10 mm. During the DS, slopes of the regression lines were lower for wet rice (slope =5.2; R2=0.88) than for dry rice (slope =6.0; R2=0.94) and maize (slope =5.5; R2=0.91; Fig. 4d–l). Due to less frequent and shorter precipitation events during the DS, the isotopic signal of the incoming water was dominated by irrigation water with nearly constant isotopic composition during the growing period. Small precipitation events were subjected to higher evaporative loss and resulted in enriched isotopic composition during this time (Table 2).
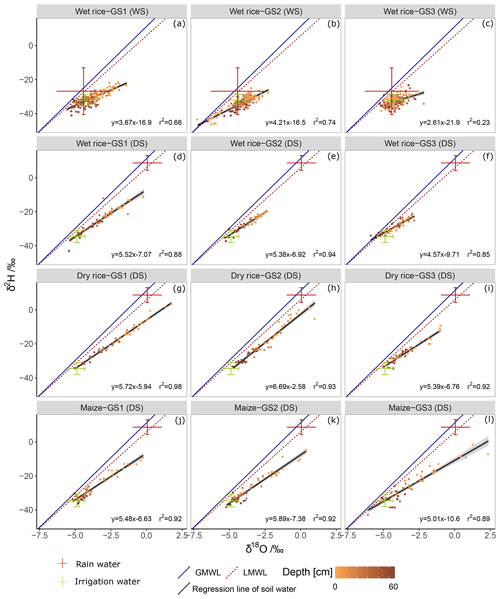
Figure 4Dual (δ18O and δ2H) isotope plots of soil water at 0–0.6 m depth, and ranges of other water sources (rainwater and irrigation water) from growing stage GS1 (a, d, g, and j), GS2 (b, e, h, and k), and GS3 (c, f, i, and l), of wet rice (a–c) during the wet season (WS), and wet rice (d–f), dry rice (g–i), and maize (j–l) during the dry season (DS) in comparison to the local meteoric water line (LMWL) and the global meteoric water line (GMWL). The grey shading represents the 95 % confidence interval of the black linear regression lines.
3.2 Fraction of evaporation loss from soil water
The estimated fraction of evaporation FE at each soil depth was derived by means of an evaporative enrichment of heavier isotopes in the soil water. Figure 5 shows estimated FE based on both isotopes for the growing seasons (WS and DS), the growing stages (GS1–GS3), the different crops (wet rice, dry rice, and maize), and for different soil depths.
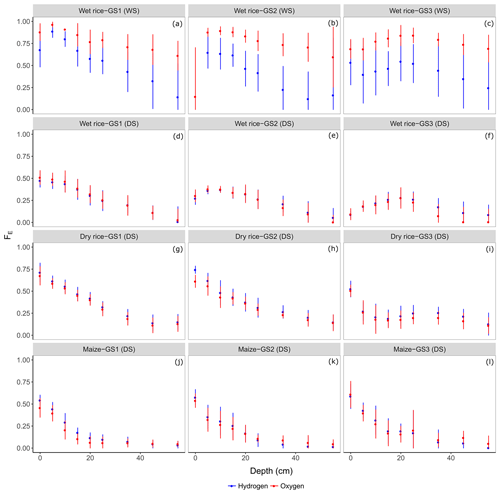
Figure 5The fraction of evaporation loss (FE; Eq. 1) estimated from δ18O and δ2H for the three main growing stages GS1 (a, d, g, and j), GS2 (b, e, h, and k), and GS3 (c, f, i, and l) of wet rice (a–c) during the wet season (WS) and wet rice (d–f), dry rice (g–i), and maize (j–l) during the dry season (DS). Mean values at each depth (0–0.6 m) are displayed with ±standard deviations.
A clear trend of FE with soil depth can be depicted at all growing stages during the DS for both crops, namely maize and dry rice, reaching below 0.2 for dry rice and even below 0.1 for maize in deep soils (Fig. 5g–l). During the DS, soils in dry rice fields showed high soil FE at shallow depths at the beginning of the first two growing stages, namely GS1 (0.54±0.1) and GS2 (0.50±0.1), which decreased to FE of around 0.27±0.1 at GS3. Furthermore, we observed lower FE average values (0.2±0.1) in deep soils between 0.25 and 0.6 m in these fields. For maize, FE remained stable at 0.3±0.1 in shallow soils throughout the season and decreased with depth for both isotopes (to around 0.07±0.05; Fig. 5j, k, and l). The FE in shallow soils of wet rice in the DS ranged from 0.42±0.08 to 0.20±0.08 (similar for both isotopes) and remained nearly stable in deep soils at 0.13±0.1 (Fig. 5d, e, and f). Overall, we did not find a similar decreasing trend with depth, as reported for dry rice and maize. Instead, particularly during GS2 and GS3, the highest fractions of FE were found at moderate soil depths from 0.1 to 0.2 m. Results regarding the estimation of FE based on δ2H and δ18O are fairly similar for all dry season crops. For wet rice in the WS, FE of both isotopes differed significantly from 0.1 to 0.2 for the topsoil and even 0.5 for deep soils (Fig. 5a, b, and c). The estimation of δp as the weighted average of the isotopic signals from large precipitation events (resulting in −36.6 ‰ for δ2H and −5.8 ‰ for δ18O) introduces a significantly higher degree of uncertainty in the estimation of FE. The isotopic composition of atmospheric vapour (δA) and relative humidity influence δ* (Eq. 2) and, therefore, also FE. The isotopic composition of δA was calculated assuming equilibrium with precipitation (Eq. 4 in the Supplement). A more depleted atmospheric moisture during the wet season tends to increase the estimated value of FE. During the WS, FE in shallow soil decreased from around 0.72±0.1 (GS1) to 0.47±0.06 (GS3) for δ2H and from 0.87±0.07 (GS1) to 0.76±0.07 (GS3) for δ18O. The general trend of slightly higher FE at moderate soil depths and again decreasing FE further down, which we observed for wet rice during the dry season, was also confirmed for wet rice during the wet season. The soil water in wet rice fields during the WS carried a larger signal of high evaporation losses down along the soil profile. The estimated FE from ponding surface water (data not shown in Fig. 5) was found to be larger during the WS than during the DS with no significant difference between δ2H and δ18O. The FE of ponded water during the WS did not fluctuate with time, and remained close to 0.92±0.07, while during the DS values decreased from GS1 (0.67±0.03) to GS3 (0.24±0.01). Here, FE of ponded surface water indicates a high evaporation loss during the WS. The evaporation signal is carried to deeper layers by subsequent infiltration and percolation.
4.1 General mechanisms in soil water movement
In the absence of lateral water transfer and assuming negligible fractionation from root water uptake, the isotopic profiles in soil water reflect a balance between mixing from infiltration, percolation, and fractionation from soil evaporation (Hsieh et al., 1998; Barnes and Allison, 1984). Depending on the evaporation effect on soil water isotopic composition and water transport processes, we found a change in the isotopic composition at around 0.2 m below the surface at our study site. This phenomenon has developed predominantly due to the existence of the dense, less permeable plough pan which separates the puddled shallow soil and non-puddled subsoil in paddy fields. The plough pan is a result of repeated ploughing and puddling over many years due to cultivation (Chen and Liu, 2002). The isotopic profiles we observed are a response to three major mechanisms that drive soil water movement at our sites, i.e. (1) matrix flow, (2) preferential flow, and (3) evaporation. These three mechanisms will be discussed in the following sections.
4.1.1 Matrix flow
In the unsaturated zone in dry rice and maize fields, the diffusive vapour transport process is dominant (Bittelli et al., 2008). Kinetic fractionation leads to the accumulation of heavy water molecules (formed by 2H and 18O) at the water–air interface, which are subsequently transported downwards and then mixed with the soil matrix (Horita et al., 2008). Downward water movement at steady-state or slowly changing conditions results in an exponential evaporation profile with depth during the drying stage that is comparable to those found in soils beneath dry rice and maize (Fig. 3i, j, m, and n; Zimmermann et al., 1966; Barnes and Allison, 1988; Rothfuss et al., 2015).
Under flooded conditions of wet rice, water slowly percolates from the ponding, which is an open water body. The upper soil layer is affected by isotopically enriched water via a gravity-driven, piston-like matrix flow. The enrichment of the heavier isotopes of soil water increases with depth (until it reaches the most enriched culmination point; Fig. 3a, b, e, and f). We assume that this is a result of the successive displacement of pre-existing mobile soil water by infiltrating water. During ponding, infiltration modifies the soil water isotopic composition in the uppermost part of the profile and re-evaporation of infiltrated water occurs. Still, soil water in fine pores represents a quasi-stationary storage, exchanging water and isotopes with the mobile phase (Gazis and Feng, 2004). As a result, the isotopic composition indicates a depletion until reaching a stable value below approximately 0.2 m. Baram et al. (2013) found a similar isotopic pattern in clay soil in Israel which they explained with a gravity-driven, piston-like matrix flow under continuous ponded infiltration.
In soils with fine pores, capillary rise could have further affected the observed isotopic patterns, depending also on depth to groundwater. It has been shown that the capillary rise of shallow groundwater can influence soil moisture and its isotopic composition in the upper metre of clayey soil (Baram et al., 2013; Clark and Fritz, 1997). An upward matrix flow through capillary rise has probably occurred in our system as well, given the fine texture of the soils (Table 1). However, the effect seems to be negligible as the groundwater signatures we measured were more enriched than the soil water found at greater soil depths (Fig. 3). This is probably due to the fact that groundwater head levels were often substantially below the deepest soil layer we sampled (Mahindawansha et al., 2018a).
The observed isotopic signals in the shallow soils could also indirectly be explained by a transpiration bias. Transpiration decreases the soil moisture but preserves the isotopic composition (Baram et al., 2013). With decreasing soil moisture, incoming water has a relatively stronger imprint on the soil's isotopic composition. Furthermore, hydraulic redistribution of water in the vadose zone is an important process of passive transport of soil water along a hydraulic gradient through the root system (Richards and Caldwell, 1987). Therefore, hydraulic redistribution can influence the pore water stable isotopic composition and reshape the soil water isotopic profile. Sprenger et al. (2016) discussed the significance of hydraulic redistribution in the soil hydrological cycle. While the review of Walter (2010) only indicated a limited impact of hydraulic redistribution on the isotopic composition of soil water, the selective removal of water combined with redistribution can be relevant. Still, isotopic measurements alone are not sufficient to estimate redistribution volumes (Emerman and Dawson, 1996), and therefore the potential impact of hydraulic redistribution requires a combination of physical transport modelling and isotopic composition and should be the focus of further studies.
4.1.2 Preferential flow through desiccation cracks
Desiccation cracks in maize fields (below 0.2 m) reached deeper (∼0.2 m) and were narrower (∼0.02 m) than those that developed in dry rice fields (own observation). We assume that this feature controls the development of soil water isotopic composition in the uppermost soil compartment. This isotopic signal is then carried to greater soil depth via leaching. At greater depth, mixing of soil water with water transported through cracks may occur. The concept of preferential transport and subsequent mixing transfers the isotopic signal of evaporation to a greater depth. Open desiccation cracks can also allow for diffusive water transport to crack surfaces at greater depth. Baram et al. (2012) observed that naturally formed desiccation crack systems can create preferential flow paths that reach more than a metre deep. In our maize fields, we observed that the isotopic composition of groundwater is strongly influenced by irrigation water, suggesting the existence of fast flow conduits (Mahindawansha et al., 2018a). He et al. (2017) have also observed leaching losses of water and nutrients in a lysimeter experiment, which they attributed to crack flow mechanisms at the same study site. Preferential flow through desiccation cracks is therefore likely to be a dominant flow pathway in rice-based cropping systems, especially for crops grown in the dry season that are planted to replace water-demanding wet rice. In line with this concept, we have recorded a gradual isotopic depletion in deep soils of dry rice and maize fields. We attribute this to subsurface mixing of isotopically enriched soil water with the depleted irrigation water from the nearby reservoir that percolated into the deep vadose zone via preferential flow paths (Baram et al., 2012; Nativ et al., 1995).
4.1.3 Evaporation effect
Evaporation and the lc excess
Systematic isotopic depletion and increasingly negative values of lc excess profiles indicated declining evaporation from GS1 to GS3 in rice (Fig. 3), particularly in shallow soils. In both dry and wet rice, the isotopic profiles showed a clear shift from more to less enriched values across the growth stages, especially in shallow soils and regardless of the season. However, the most isotopically enriched water in wet rice was transferred down to even greater depths in conjunction with plant growth (Fig. 3a, b, e, and f). In maize fields, the evaporation fraction gradually increased towards the end of the season when irrigation ceased (Figs. 2 and 3), resulting in dry soil conditions and a soil water deficit. Therefore, we conclude that there is an influence of the crop type and growth stage on evaporation fractionation in the soil water, matching previous reports that the plant cover reduces kinetic fractionation processes in the soils (Burger and Seiler, 1992; Dubbert et al., 2013).
Evaporation and the LMWL
Comparisons of regression lines of soil water samples to the GMWL in the dual isotope space (δ18O and δ2H) helped to identify the environmental conditions during soil evaporation with regards to season and crop (Fig. 4). The slope of the δ18O–δ2H relationship decreases because of kinetic fractionation (Dansgaard, 1964). This deviation can be used to estimate evaporation losses (Clark and Fritz, 1997). The steeper slopes of the dry soils (maize and dry rice) can be explained by an increase in the effective thickness of the vapour transport layer (Barnes and Allison, 1988) compared to the soils of wet rice. For soils under wet rice, a steeper gradient near the surface was found, similar to observations regarding saturated soils made by Allison (1982). During the WS, the δ18O–δ2H relationship of deep soil water under wet rice fell even further below the LMWL from GS2 to GS3 (Fig. 4b and c). In contrast, shallow soils plotted closer to the LMWL indicated lower evaporation rates. Furthermore, deep soil water showed isotopic similarities with the irrigation water. Following these observations, we assume that the deep soil isotopic profiles result from a mixing of soil water with irrigation water, with the latter likely stemming from the previous DS (memory of the old isotopic signal) that moved downward via matrix flow. Due to the low rates of percolation of 1 to 5 mm d−1 in clay paddy soils (Bouman and Tuong, 2001), deep soil profiles with multiple soil layers may reveal a record of antecedent evaporation conditions or preferential flow shortcuts between the layers.
Apart from this, all soil profiles presented enriched values and distinct evaporation processes during the WS (Fig. 4a–c). Lower slopes of evaporation lines in wet soil compared to dry soil point to greater kinetic effects (Cooper et al., 1991). Slopes of evaporation lines < 3.5 were reported to indicate diffusion processes (Allison et al., 1983). We, therefore, assume that diffusion processes in the subsurface were relevant for shaping soil isotopic profiles in the WS, especially in GS1 and GS3 (Fig. 4a and c). During GS2, mixing processes between infiltrating water dominated and limited diffusion processes due to continuous intense precipitation events during that time. In line with this, a higher correlation between plant water (xylem) and rainwater during this time, compared to the other growing stages, was reported by Mahindawansha et al. (2018b). Overall, an enrichment of soil water isotopic composition during the WS and a depletion during the DS is comparable to observations made by Hsieh et al. (1998) in an arid to humid transect in Hawaii. Similar differences between depleted winter and enriched summer isotopic profiles in combination with mixing processes were also reported by Baram et al. (2013) and DePaolo et al. (2004).
Unproductive water losses via evaporation
Kinetic fractionation and its imprint on soil water isotopic profiles in the shallow soil is relatively small in tropical climates given the generally high relative humidity (Gonfiantini, 1986). Nevertheless, our observations point to kinetic fractionation down to a depth of ∼0.2 m, which is shallower than the average depth in temperate regions (∼0.3 m; Gazis and Feng, 2004; Sutanto et al., 2012), the Mediterranean (∼0.5 m; Oshun et al., 2016; Simonin et al., 2014), or in arid climates (∼3 m; Allison and Hughes, 1983; Singleton et al., 2004). Shallow soils exhibit a decreasing trend of FE during both WS and DS from the beginning of the growing season towards its end (Fig. 5), which is most likely driven by an increase in the leaf area of the above-ground vegetation. Rothfuss et al. (2010) made comparable observations in a laboratory-based experiment on soil columns and reported changes in FE over time. They found values starting with 100 % at bare soil conditions and dropping to 5 % at the full development of the deep-rooting perennial grass grown in the columns. In our study, the fraction of soil evaporation was estimated to be 40 % at the beginning of the DS and decreased to 25 % towards the end, while it dropped from 80 % to 60 % during the WS. Values of about 30 % (maize) and 50 % (dry rice) for evaporation losses were reported in Asia at the same sites in the Philippines, based on eddy covariance measurements by Alberto et al. (2014). Similar values were confirmed by Bouman et al. (2005), based on a review of tropical upland and lowland rice varieties under irrigated aerobic conditions. Simpson et al. (1992) reported 40 % for flooded rice fields in a semi-arid region of southeastern Australia and Maruyama, and Kuwagata (2010) reported about 60 % for paddy fields in southwestern Japan. During the WS, however, FE was higher in the shallow soil compared to the DS. This finding might be related to the high temperatures leading to higher water pressure deficits between soil water and the atmosphere. The substantially large difference in FE during the WS between δ2H- and δ18O-based assessments can be related to the different hydrogen compounds. The values we obtained refer to the fraction of water loss from the soil matrix and small/intermediate pores. With isotope methods, we only estimated unproductive evaporation losses from the soil. However, Wei et al. (2018) showed that an isotopic approach can also lead to higher estimates of the fractions compared to model results for rice and maize in Tsukuba, Japan. Overall, we conclude that the isotope method provides comparable results to previous studies.
4.2 Differences in fractionation of δ2H and δ18O
Apart from the highly depleted isotopic signal for δ2H observed in deep soil under wet rice fields during the WS (Fig. 3b), there was a systematic deviation of about 20 % between δ2H and δ18O fractionation in shallow soil and 40 % in deep soil (Fig. 5a–c). This difference may have resulted from the formation of specific hydrogen compounds under continuous inundation conditions. Flooding affects soils chemically, physically, and biologically, resulting in a reduction of redox potential (Fageria et al., 2011; Zhang et al., 2015). Due to the anaerobic conditions that develop in submerged soil, hydrogen compounds such as CH4, H2S, H2, and can be produced via microbial anaerobic respiration (Fageria et al., 2011; Gerardi, 2003). The formation of these hydrogen compounds leads to isotopic exchange and bias in δ2H, as observed by Baram et al. (2013) in clay soils below ponded wastewater conditions. CH4 emissions in wet rice fields on our study site were higher during the WS compared to the DS (Weller et al., 2016), and this may have caused lower slopes in the dual isotope plots observed (Fig. 4a–c).
Furthermore, the equilibrium constant for isotopic partitioning of liquid water with vapour (1000 lnα) is a function of the temperature (here we present the values at 27 ∘C) and the sign of the value (positive), for example, H2O(l)↔H2O(g) for δ18O +9.2 (Freidman and O'Neil, 1977; Majoube, 1971) and +74.3 for δ2H (Majoube, 1971). Water vapour δ2H further isotopically fractionates with CH4(g) (1000 ln; see Bottinga, 1969), H2S(g) (1000 ln as in Galley et al., 1972; Clark and Fritz, 1997), and liquid water with CH4(g) with 1000 ln (Horibe and Craig, 1995), leading to higher δ2H (enriched) in both phases. Moreover, liquid water and water vapour further manifest an equilibrium with H2(g) with higher equilibrium fractionation (Bottinga, 1969; Rolston et al., 1976). As a result, the assumption of δ2H enrichment is further reinforced. The difference between δ2H and δ18O has been found to be more pronounced at a greater depth, suggesting the formation of hydrogen compounds in deeper soil (Fig. 5a–c). Besides, exchange rates and fractionation with kaolinite and smectite (Gilg and Sheppard, 1996) are faster and more pronounced for δ2H. The assumption of this dissimilarity between δ2H and δ18O can be quantified by a sensitivity analysis, giving a relative depletion by 5±2 ‰ of δ2H. Because of the above processes, bias can result in the calculation of FE during the WS. Due to the high standard deviation of the isotopic composition in extreme precipitation events during the WS, prediction of the original water source at a given time was also more uncertain. The FE values are sensitive to the isotopic composition of atmospheric vapour and original water input. Nevertheless, only seasonal averages were assigned in the calculation. This difference was not prominent in wet rice fields under anaerobic conditions during the DS, where oxidizing conditions occurred in time gaps between irrigation events; it was also not observed in dry rice and maize fields.
In addition, vacuum-extracted soil water also contains bound water plus adsorbed water, making isotopic composition lower (Gaj et al., 2017; Velde, 1992) and separate from additional systematic errors resulting from the extraction method (Orlowski et al., 2016). High water-holding capacity (Brouwer et al., 2001; Hazelton and Murphy, 2016) and the shrinking and swelling behaviour (Baram et al., 2013; Dasog et al., 1988) of clayey soil add complexity to the analysis. Determination of αk can also result in estimation errors (i.e. a maximum uncertainty of the partitioning) of 1 % to 29 %, depending on the value of αk and the day of the partitioning (Rothfuss et al., 2010).
We identified three main processes which are responsible for variations in the soil water isotopic profile, namely soil evaporation, slow soil water movement via matrix flow, and the refilling of deep soil water through preferential flows via desiccation cracks. Apart from this, we also quantified the relative fraction of soil water returning to the atmosphere as direct evaporation as unproductive soil water losses and related its pattern to crop types and seasons. However, independent tools to confirm the findings of complex soil water isotope studies on evaporation would be needed. There was a clear isotopic separation between shallow and deep soil, with higher enrichment in shallow soil at around 0.2 m below the surface. Deep soil in wet rice fields often presented inverted evaporation profiles because deep soil layers carried over the history of the transported evaporation signal from the previous season. Shallow soils in maize fields showed a stronger soil evaporation effect than rice fields. Compared to the original water input, greater water loss was estimated during the WS compared to the DS when referring to evaporation from the soil matrix. The observation of the difference in the fractionation of δ2H and δ18O deserves further research. Even though we provided a theoretical background of how this fractionation might occur, we were not able to measure all the different components which affect the fractionation. Further research into these processes would help to better understand the evaporation process.
To conclude, water losses via soil evaporation are a major unproductive loss next to leaching losses, especially during the early growing stage. Therefore, our study helps to increase understanding of soil water transport processes and evaporation losses from soil in response to crop rotation systems. Our hypothesis of reducing the unproductive water losses by introducing dry seasonal crops is supported by isotope data. Farmers should apply mitigation methods to reduce soil water evaporation, for example, by mulching or growing cover crops in the fallow period and by protecting the plough pan.
The data that support the findings of this study are available upon request from the corresponding author.
The supplement related to this article is available online at: https://doi.org/10.5194/hess-24-3627-2020-supplement.
AM conducted the field sampling, laboratory analysis, and data visualization and prepared the original draft. AM, CK, and LB contributed to the data interpretation. CK, PK, and LB assisted with the review and editing of the paper.
The authors declare that they have no conflict of interest.
This article is part of the special issue “Water, isotope and solute fluxes in the soil–plant–atmosphere interface: investigations from the canopy to the root zone”. It is not associated with a conference.
This research was undertaken as part of the ICON project phase II (“Introducing Non-Flooded Crops in Rice-Dominated Landscapes: Impact on Carbon, Nitrogen and Water Cycles”) under Subproject SP07 (“Monitoring and modeling of water and related nutrient fluxes in rice-based cropping systems”). We acknowledge the support of the International Rice Research Institute in the Philippines and, in particular, Reiner Wassmann for his support. We gratefully acknowledge the technical support from Heathcliff Racela during the experiments. We would like to thank Samantha Serratore for editing the final paper.
This research has been supported by the Deutsche
Forschungsgemeinschaft (DFG) (Research Unit FOR1701; grant no. BR2238/9-2).
This open-access publication was funded
by Justus Liebig University Giessen.
This paper was edited by Matthias Sprenger and reviewed by Matthias Beyer and two anonymous referees.
Alberto, Ma. C. R., Quilty, J. R., Buresh, R. J., Wassmann, R., Haidar, S., Correa, T. Q., and Sandro, J. M.: Actual evapotranspiration and dual crop coefficients for dry-seeded rice and hybrid maize grown with overhead sprinkler irrigation, Agr. Water Manage., 136, 1–12, https://doi.org/10.1016/j.agwat.2014.01.005, 2014.
Allison, G. B.: The relationship between 18O and deuterium in water in sand columns undergoing evaporation, J. Hydrol., 55, 163–169, 1982.
Allison, G. B. and Hughes, M. W.: The use of natural tracers as indicators of soil-water movement in a temperate semi-arid region, J. Hydrol., 60, 157–173, 1983.
Baram, S., Kurtzman, D., and Dahan, O.: Water percolation through a clayey vadose zone, J. Hydrol., 424–425, 165–171, https://doi.org/10.1016/j.jhydrol.2011.12.040, 2012.
Baram, S., Ronen, Z., Kurtzman, D., Külls, C., and Dahan, O.: Desiccation-crack-induced salinization in deep clay sediment, Hydrol. Earth Syst. Sci., 17, 1533–1545, https://doi.org/10.5194/hess-17-1533-2013, 2013.
Barnes, C. J. and Allison, G. B.: The distribution of deuterium and 18O in dry soils: 1. Theory, J. Hydrol., 60, 141–156, https://doi.org/10.1016/0022-1694(83)90018-5, 1983.
Barnes, C. J. and Allison, G. B.: The distribution of deuterium and 18O in dry soils: 3. Theory for non-isothermal water movement, J. Hydrol., 74, 119–135, https://doi.org/10.1016/0022-1694(84)90144-6, 1984.
Barnes, C. J. and Allison, G. B.: Tracing of water movement in the unsaturated zone using stable isotopes of hydrogen and oxygen, J. Hydrol., 100, 143–176, https://doi.org/10.1016/0022-1694(88)90184-9, 1988.
Barnes, C. J. and Turner, J. V.: Isotopic Exchange in Soil Water, in Isotope Tracers in Catchment Hydrology, chap. 5, edited by: Kendall, C. and McDonnell, J. J., Elsevier, Amsterdam, 137–163, 1998.
Belder, P., Bouman, B. A. M., Cabangon, R., Guoan, L., Quilang, E. J. P., Yuanhua, L., Spiertz, J. H. J., and Tuong, T. P.: Effect of water-saving irrigation on rice yield and water use in typical lowland conditions in Asia, Agr. Water Manage., 65, 193–210, https://doi.org/10.1016/j.agwat.2003.09.002, 2004.
Benettin, P., Volkmann, T. H. M., von Freyberg, J., Frentress, J., Penna, D., Dawson, T. E., and Kirchner, J. W.: Effects of climatic seasonality on the isotopic composition of evaporating soil waters, Hydrol. Earth Syst. Sci., 22, 2881–2890, https://doi.org/10.5194/hess-22-2881-2018, 2018.
Beyer, M., Koeniger, P., Gaj, M., Hamutoko, J. T., Wanke, H., and Himmelsbach, T.: A deuterium-based labeling technique for the investigation of rooting depths, water uptake dynamics and unsaturated zone water transport in semiarid environments, J. Hydrol., 533, 627–643, https://doi.org/10.1016/j.jhydrol.2015.12.037, 2016.
Bittelli, M., Ventura, F., Campbell, G. S., Snyder, R. L., Gallegati, F., and Pisa, P. R.: Coupling of heat, water vapor, and liquid water fluxes to compute evaporation in bare soils, J. Hydrol., 362, 191–205, https://doi.org/10.1016/j.jhydrol.2008.08.014, 2008.
Bottinga, Y.: Calculated fractionation factors for carbon and hydrogen isotope exchange in the system calcite-carbon dioxide-graphite-methane-hydrogen-water vapor, Geochim. Cosmochim. Ac., 33, 49–64, https://doi.org/10.1016/0016-7037(69)90092-1, 1969.
Bouman, B. A. M.: Water Management in Irrigated Rice: Coping with Water Scarcity, Int. Rice Res. Inst., Los Banos, available at: http://books.irri.org/9789712202193_content.pdf (last access: 7 August 2018), 2007.
Bouman, B. A. M. and Tuong, T. P.: Field water management to save water and increase its productivity in irrigated lowland rice, Agr. Water Manage., 49, 11–30, 2001.
Bouman, B. A. M., Peng, S., Castañeda, A. R., and Visperas, R. M.: Yield and water use of irrigated tropical aerobic rice systems, Agr. Water Manage., 74, 87–105, https://doi.org/10.1016/j.agwat.2004.11.007, 2005.
Braud, I., Biron, P., Bariac, T., Richard, P., Canale, L., Gaudet, J. P., and Vauclin, M.: Isotopic composition of bare soil evaporated water vapor. Part I: RUBIC, J. Hydrol., 369, 1–16, https://doi.org/10.1016/j.jhydrol.2009.01.034, 2009.
Brouwer, C., Prins, K., Kay, M., and Heibloem, M.: Irrigation Water Management: Irrigation Methods, Training manual, FAO – Food and Agriculture Organization of the united nations, Via delle Terme di Caracalla, 00100 Rome, Italy, available at: http://www.fao.org/docrep/s8684e/s8684e00.htm#Contents (last access: 10 June 2020), 2001.
Burger, H. M. and Seiler, K. P.: Evaporation from soil water under humid climate conditions and its impact on deuterium and 18O concentrations in groundwater, Isot. Tech. Water Resour. Dev. 1991, available at: http://inis.iaea.org/Search/search.aspx?orig_q=RN:23034850 (last access: 8 November 2018), 1992.
Chen, S.-K. and Liu, C. W.: Analysis of water movement in paddy rice fields (I) experimental studies, J. Hydrol., 260, 206–215, https://doi.org/10.1016/S0022-1694(01)00615-1, 2002.
Clark, I. D. and Fritz, P.: Environmental Isotopes in Hydrogeology, CRC Press, Boca Raton, 1997.
Cooper, L. W., DeNiro, M. J., and Keeley, J. E.: The relationship between stable oxygen and hydrogen isotope ratios of water in astomatal plants, available at: https://www.geochemsoc.org/files/5414/1269/7660/SP-3_247-256_Cooper.pdf (last access: 10 July 2020), 247–255, 1991.
Counce, P. A., Keisling, T. C., and Mitchell, A. J.: A Uniform, Objective, and Adaptive System for Expressing Rice Development, Crop Sci., 40, 436–443, https://doi.org/10.2135/cropsci2000.402436x, 2000.
Craig, H. and Gordon, L. I.: Deuterium and oxygen 18 variations in the ocean and the marine atmosphere, in: Stable Isotopes in Oceanographic Studies and Paleotemperatures, edited by: Tongiorgi, E., Stable Isotopes in Oceanographic Studies and Paleotemperatures, Spoleto, Italy, Laboratorio di Geologica Nucleare, Pisa, Italy, 9–130, 1965.
Daly, E., Porporato, A., and Rodriguez-Iturbe, I.: Coupled Dynamics of Photosynthesis, Transpiration, and Soil Water Balance. Part I: Upscaling from Hourly to Daily Level, J. Hydrometeorol., 5, 546–558, https://doi.org/10.1175/1525-7541(2004)005<0546:CDOPTA>2.0.CO;2, 2004.
Dansgaard, W.: Stable isotopes in precipitation, Tellus, 16, 436–468, https://doi.org/10.3402/tellusa.v16i4.8993, 1964.
Dasog, G. S., Acton, D. F., Mermut, A. R., and Jong, E. D.: Shrink-swell potential and cracking in clay soils of Saskatchewan, Can. J. Soil Sci., 68, 251–260, 1988.
DePaolo, D. J., Conrad, M. E., Maher, K., and Gee, G. W.: Evaporation Effects on Oxygen and Hydrogen Isotopes in Deep Vadose Zone Pore Fluids at Hanford, Washington, Vadose Zone J., 3, 220–232, https://doi.org/10.2136/vzj2004.2200, 2004.
Dubbert, M., Cuntz, M., Piayda, A., Maguás, C., and Werner, C.: Partitioning evapotranspiration – Testing the Craig and Gordon model with field measurements of oxygen isotope ratios of evaporative fluxes, J. Hydrol., 496, 142–153, https://doi.org/10.1016/j.jhydrol.2013.05.033, 2013.
Emerman, S. H. and Dawson, T. E.: Hydraulic lift and its influence on the water content of the rhizosphere: an example from sugar maple, Acer saccharum, Oecologia, 108, 273–278, 1996.
Fageria, N. K., Carvalho, G. D., Santos, A. B., Ferreira, E. P. B., and Knupp, A. M.: Chemistry of Lowland Rice Soils and Nutrient Availability, Commun. Soil Sci. Plant Anal., 42, 1913–1933, https://doi.org/10.1080/00103624.2011.591467, 2011.
FAO: Save and Grow in Practice: Maize, Rice, Wheat ?a Guide to Sustainable Cereal Production, in Save and Grow in practice: maize, rice, wheat?: a guide to sustainable cereal production, p. 124, 2016.
Freidman, T. and O'Neil, J. R.: Compilation of stable isotope fractionation factors of geochemical interest: US Geol, Surv. Prof Pap., 440, 1977.
Gaj, M., Beyer, M., Koeniger, P., Wanke, H., Hamutoko, J., and Himmelsbach, T.: In situ unsaturated zone water stable isotope (2H and 18O) measurements in semi-arid environments: a soil water balance, Hydrol. Earth Syst. Sci., 20, 715–731, https://doi.org/10.5194/hess-20-715-2016, 2016.
Gaj, M., Kaufhold, S., Koeniger, P., Beyer, M., Weiler, M., and Himmelsbach, T.: Mineral mediated isotope fractionation of soil water: Mineral mediated isotope fractionation of soil water, Rapid Commun. Mass Sp., 31, 269–280, https://doi.org/10.1002/rcm.7787, 2017.
Galley, M. R., Miller, A. I., Atherley, J. F., and Mohn, M.: GS process physical properties, Atomic Energy of Canada Ltd., 1972.
Gangi, L., Rothfuss, Y., Ogée, J., Wingate, L., Vereecken, H., and Brüggemann, N.: A New Method for In Situ Measurements of Oxygen Isotopologues of Soil Water and Carbon Dioxide with High Time Resolution, Vadose Zone J., 14, 1–14, https://doi.org/10.2136/vzj2014.11.0169, 2015.
Gat, J. R.: Oxygen and Hydrogen Isotopes in the Hydrologic Cycle, Annu. Rev. Earth Planet. Sci., 24, 225–262, https://doi.org/10.1146/annurev.earth.24.1.225, 1996.
Gazis, C. and Feng, X.: A stable isotope study of soil water: evidence for mixing and preferential flow paths, Geoderma, 119, 97–111, https://doi.org/10.1016/S0016-7061(03)00243-X, 2004.
Gerardi, M. H.: The Microbiology of Anaerobic Digesters, John Wiley & Sons, Hoboken, New Jersey, 2003.
GNIP-IAEA: International Atomic Energy Agency (IAEA) WISER – GNIP, available at: https://nucleus.iaea.org/wiser/gnip.php?ll_latlon=&ur_latlon=&country=&wmo_region=&date_start=1953&date_end=201&iso_o18=on&iso_h2=on&action=Search (last access: 16 August 2017), 2016.
Gibson, J. J., Birks, S. J., and Yi, Y.: Stable isotope mass balance of lakes: a contemporary perspective, Quaternary Sci. Rev., 131, 316–328, https://doi.org/10.1016/j.quascirev.2015.04.013, 2016.
Gilg, H. A. and Sheppard, S. M.: Hydrogen isotope fractionation between kaolinite and water revisited, Geochim. Cosmochim. Ac., 60, 529–533, 1996.
Gonfiantini, R.: Environmental isotopes in lake studies, Handb. Environ. Isot. Geochem. Terr. Environ., 2, 113–168, 1986.
Good, S. P., Soderberg, K., Guan, K., King, E. G., Scanlon, T. M., and Caylor, K. K.: δ2H isotopic flux partitioning of evapotranspiration over a grass field following a water pulse and subsequent dry down, Water Resour. Res., 50, 1410–1432, https://doi.org/10.1002/2013WR014333, 2014.
Hazelton, P. and Murphy, B.: Interpreting Soil Test Results: What Do All the Numbers Mean?, CSIRO Publishing, available at: https://www.publish.csiro.au/book/7386/ (last access: 22 November 2018), 2016.
He, Y., Siemens, J., Amelung, W., Goldbach, H., Wassmann, R., Alberto, Ma, C. R., Lücke, A., and Lehndorff, E.: Carbon release from rice roots under
paddy rice and maize–paddy rice cropping, Agr. Ecosyst. Environ., 210, 15–24, https://doi.org/10.1016/j.agee.2015.04.029, 2015.
He, Y., Lehndorff, E., Amelung, W., Wassmann, R., Alberto, Ma. C., von Unold, G., and Siemens, J.: Drainage and leaching losses of nitrogen and dissolved organic carbon after introducing maize into a continuous paddy-rice crop rotation, Agr. Ecosyst. Environ., 249, 91–100, https://doi.org/10.1016/j.agee.2017.08.021, 2017.
Heinz, E., Kraft, P., Buchen, C., Frede, H.-G., Aquino, E., and Breuer, L.: Set Up of an Automatic Water Quality Sampling System in Irrigation Agriculture, Sensors, 14, 212–228, https://doi.org/10.3390/s140100212, 2013.
Horibe, Y. and Craig, H.: DH fractionation in the system methane-hydrogen-water, Geochim. Cosmochim. Ac., 59, 5209–5217, https://doi.org/10.1016/0016-7037(95)00391-6, 1995.
Horita, J., Rozanski, K., and Cohen, S.: Isotope effects in the evaporation of water: a status report of the Craig–Gordon model, Isotopes Environ. Health Stud., 44, 23–49, https://doi.org/10.1080/10256010801887174, 2008.
Hsieh, J. C. C., Chadwick, O. A., Kelly, E. F., and Savin, S. M.: Oxygen isotopic composition of soil water: Quantifying evaporation and transpiration, Geoderma, 82, 269–293, https://doi.org/10.1016/S0016-7061(97)00105-5, 1998.
Janssen, M. and Lennartz, B.: Horizontal and vertical water and solute fluxes in paddy rice fields, Soil Till. Res., 94, 133–141, https://doi.org/10.1016/j.still.2006.07.010, 2007.
Kendall, C. and Caldwell, E.: Fundamentals of isotope geochemistry, in Isotope Tracers in Catchment Hydrology, Elsevier, 51–86, 1999.
Kool, D., Agam, N., Lazarovitch, N., Heitman, J. L., Sauer, T. J., and Ben-Gal, A.: A review of approaches for evapotranspiration partitioning, Agr. Forest Meteorol., 184, 56–70, https://doi.org/10.1016/j.agrformet.2013.09.003, 2014.
Kudo, Y., Noborio, K., Shimoozono, N., and Kurihara, R.: The effective water management practice for mitigating greenhouse gas emissions and maintaining rice yield in central Japan, Agr. Ecosyst. Environ., 186, 77–85, https://doi.org/10.1016/j.agee.2014.01.015, 2014.
Kutilek, M. and Nielsen, D. R.: Soil hydrology: texbook for students of soil science, agriculture, forestry, geoecology, hydrology, geomorphology and other related disciplines, Soil Hydrol. Texbook Stud. Soil Sci. Agric. For. Geoecology Hydrol. Geomorphol. Relat. Discip., 370 pp., 1994.
Landwehr, J. M. and Coplen, T. B.: Line-conditioned excess: a new method for characterizing stable hydrogen and oxygen isotope ratios in hydrologic systems, in International Conference on Isotopes in Environmental Studies, Int. At. Energy Agency Vienna, 132–135, 2006.
Liu, C., Zhang, X., and Zhang, Y.: Determination of daily evaporation and evapotranspiration of winter wheat and maize by large-scale weighing lysimeter and micro-lysimeter, Agr. For. Meteorol., 111, 109–120, https://doi.org/10.1016/S0168-1923(02)00015-1, 2002.
Liu, Y., Liu, F., Xu, Z., Zhang, J., Wang, L., and An, S.: Variations of soil water isotopes and effective contribution times of precipitation and throughfall to alpine soil water, in Wolong Nature Reserve, China, CATENA, 126, 201–208, https://doi.org/10.1016/j.catena.2014.11.008, 2015.
Maclean, J. L., Dawe, D. C., and Hettel, G. P., Eds.: Rice almanac: source book for the most important economic activity on earth, 3rd ed., CABI Pub, Oxon, UK, 2002.
Mahindawansha, A., Breuer, L., Chamorro, A., and Kraft, P.: High-Frequency Water Isotopic Analysis Using an Automatic Water Sampling System in Rice-Based Cropping Systems, Water, 10, 1327, https://doi.org/10.3390/w10101327, 2018a.
Mahindawansha, A., Orlowski, N., Kraft, P., Rothfuss, Y., Racela, H., and Breuer, L.: Quantification of plant water uptake by water stable isotopes in rice paddy systems, Plant Soil, 429, 281–302, https://doi.org/10.1007/s11104-018-3693-7, 2018b.
Majoube, M.: Fractionnement en oxygène 18 et en deutérium entre l'eau et sa vapeur, J. Chim. Phys., 68, 1423–1436, https://doi.org/10.1051/jcp/1971681423, 1971.
Maruyama, A. and Kuwagata, T.: Coupling land surface and crop growth models to estimate the effects of changes in the growing season on energy balance and water use of rice paddies, Agr. Forest Meteorol., 150, 919–930, https://doi.org/10.1016/j.agrformet.2010.02.011, 2010.
Mathieu, R. and Bariac, T.: A numerical model for the simulation of stable isotope profiles in drying soils, J. Geophys. Res.-Atmos., 101, 12685–12696, https://doi.org/10.1029/96JD00223, 1996.
Mekonnen, M. M. and Hoekstra, A. Y.: The green, blue and grey water footprint of crops and derived crop products, Hydrol. Earth Syst. Sci., 15, 1577–1600, https://doi.org/10.5194/hess-15-1577-2011, 2011.
Melayah, A., Bruckler, L., and Bariac, T.: Modeling the Transport of Water Stable Isotopes in Unsaturated Soils Under Natural Conditions: 2. Comparison With Field Experiments, Water Resour. Res., 32, 2055–2065, https://doi.org/10.1029/96WR00673, 1996.
Nativ, R., Adar, E., Dahan, O., and Geyh, M.: Water Recharge and Solute Transport Through the Vadose Zone of Fractured Chalk Under Desert Conditions, Water Resour. Res., 31, 253–261, https://doi.org/10.1029/94WR02536, 1995.
Oerter, E. J. and Bowen, G.: In situ monitoring of H and O stable isotopes in soil water reveals ecohydrologic dynamics in managed soil systems, Ecohydrology, 10, e1841, https://doi.org/10.1002/eco.1841, 2017.
Orlowski, N., Frede, H.-G., Brüggemann, N., and Breuer, L.: Validation and application of a cryogenic vacuum extraction system for soil and plant water extraction for isotope analysis, J. Sens. Sens. Syst., 2, 179–193, https://doi.org/10.5194/jsss-2-179-2013, 2013.
Orlowski, N., Breuer, L., and McDonnell, J. J.: Critical issues with cryogenic extraction of soil water for stable isotope analysis, Ecohydrology, 9, 1–5, https://doi.org/10.1002/eco.1722, 2016.
Oshun, J., Dietrich, W. E., Dawson, T. E., and Fung, I.: Dynamic, structured heterogeneity of water isotopes inside hillslopes, Water Resour. Res., 52, 164–189, https://doi.org/10.1002/2015WR017485, 2016.
Pfister, S., Bayer, P., Koehler, A., and Hellweg, S.: Projected water consumption in future global agriculture: Scenarios and related impacts, Sci. Total Environ., 409, 4206–4216, https://doi.org/10.1016/j.scitotenv.2011.07.019, 2011.
Richards, J. H. and Caldwell, M. M.: Hydraulic lift: substantial nocturnal water transport between soil layers by Artemisia tridentata roots, Oecologia, 73, 486–489, 1987.
Rolston, J. H., Den Hartog, J., and Butler, J. P.: The deuterium isotope separation factor between hydrogen and liquid water, J. Phys. Chem., 80, 1064–1067, https://doi.org/10.1021/j100551a008, 1976.
Rothfuss, Y., Biron, P., Braud, I., Canale, L., Durand, J.-L., Gaudet, J.-P., Richard, P., Vauclin, M., and Bariac, T.: Partitioning evapotranspiration fluxes into soil evaporation and plant transpiration using water stable isotopes under controlled conditions, Hydrol. Process., 24, 3177–3194, https://doi.org/10.1002/hyp.7743, 2010.
Rothfuss, Y., Merz, S., Vanderborght, J., Hermes, N., Weuthen, A., Pohlmeier, A., Vereecken, H., and Brüggemann, N.: Long-term and high-frequency non-destructive monitoring of water stable isotope profiles in an evaporating soil column, Hydrol. Earth Syst. Sci., 19, 4067–4080, https://doi.org/10.5194/hess-19-4067-2015, 2015.
Rozanski, K., Araguás-Araguás, L., and Gonfiantini, R.: Isotopic Patterns in Modern Global Precipitation, in Climate Change in Continental Isotopic Records, edited by: Swart, P. K., Lohmann, K. C., Mckenzie, J., and Savin, S., American Geophysical Union, 1–36, 1993.
Simonin, K. A., Link, P., Rempe, D., Miller, S., Oshun, J., Bode, C., Dietrich, W. E., Fung, I., and Dawson, T. E.: Vegetation induced changes in the stable isotope composition of near surface humidity, Ecohydrology, 7, 936–949, https://doi.org/10.1002/eco.1420, 2014.
Simpson, H. J., Herczeg, A. L., and Meyer, W. S.: Stable isotope ratios in irrigation water can estimate rice crop evaporation, Geophys. Res. Lett., 19, 377–380, https://doi.org/10.1029/91GL02952, 1992.
Singleton, M. J., Sonnenthal, E. L., Conrad, M. E., DePaolo, D. J., and Gee, G. W.: Multiphase Reactive Transport Modeling of Seasonal Infiltration Events and Stable Isotope Fractionation in Unsaturated Zone Pore Water and Vapor at the Hanford Site, Vadose Zone J., 3, 775–785, https://doi.org/10.2136/vzj2004.0775, 2004.
Sprenger, M., Leistert, H., Gimbel, K., and Weiler, M.: Illuminating hydrological processes at the soil-vegetation-atmosphere interface with water stable isotopes, Rev. Geophys., 54, 674–704, https://doi.org/10.1002/2015RG000515, 2016.
Sprenger, M., Tetzlaff, D., and Soulsby, C.: Soil water stable isotopes reveal evaporation dynamics at the soil–plant–atmosphere interface of the critical zone, Hydrol. Earth Syst. Sci., 21, 3839–3858, https://doi.org/10.5194/hess-21-3839-2017, 2017.
Sutanto, S. J., Wenninger, J., Coenders-Gerrits, A. M. J., and Uhlenbrook, S.: Partitioning of evaporation into transpiration, soil evaporation and interception: a comparison between isotope measurements and a HYDRUS-1D model, Hydrol. Earth Syst. Sci., 16, 2605–2616, https://doi.org/10.5194/hess-16-2605-2012, 2012.
Tertre, E., Savoye, S., Hubert, F., Prêt, D., Dabat, T., and Ferrage, E.: Diffusion of Water through the Dual-Porosity Swelling Clay Mineral Vermiculite, Environ. Sci. Technol., 52, 1899–1907, https://doi.org/10.1021/acs.est.7b05343, 2018.
Timsina, J., Jat, M. L., and Majumdar, K.: Rice-maize systems of South Asia: current status, future prospects and research priorities for nutrient management, Plant Soil, 335, 65–82, https://doi.org/10.1007/s11104-010-0418-y, 2010.
Towprayoon, S., Smakgahn, K., and Poonkaew, S.: Mitigation of methane and nitrous oxide emissions from drained irrigated rice fields, Chemosphere, 59, 1547–1556, https://doi.org/10.1016/j.chemosphere.2005.02.009, 2005.
Twining, J., Stone, D., Tadros, C., Henderson-Sellers, A., and Williams, A.: Moisture Isotopes in the Biosphere and Atmosphere (MIBA) in Australia: A priori estimates and preliminary observations of stable water isotopes in soil, plant and vapour for the Tumbarumba Field Campaign, Global Planet. Change, 51, 59–72, https://doi.org/10.1016/j.gloplacha.2005.12.005, 2006.
Velde, B.: Introduction to clay minerals: chemistry, origins, uses and environmental significance., Introd. Clay Miner. Chem. Orig. Uses Environ. Significance, available at: https://www.cabdirect.org/cabdirect/abstract/19931982767 (last access: 21 January 2020), 1992.
Volkmann, T. H. M., Haberer, K., Gessler, A., and Weiler, M.: High-resolution isotope measurements resolve rapid ecohydrological dynamics at the soil–plant interface, New Phytol., 210, 839–849, https://doi.org/10.1111/nph.13868, 2016.
Walter, K.: Einfluss der Pflanzen auf die Isotopenzusammensetzung des Abflusses in Einzugsgebieten, PhD Thesis, Diplomarbeit, Institut für Hydrologie, Albert-Ludwigs-Universität Freiburg,, 2010.
Wang, Z., Ankeny, M., and Horton, R.: The impact of water vapor diodes on soil water redistribution, J. Hydrol., 552, 600–608, https://doi.org/10.1016/j.jhydrol.2017.07.009, 2017.
Wei, Z., Yoshimura, K., Okazaki, A., Kim, W., Liu, Z., and Yokoi, M.: Partitioning of evapotranspiration using high-frequency water vapor isotopic measurement over a rice paddy field: Partitioning of evapotranspiration, Water Resour. Res., 51, 3716–3729, https://doi.org/10.1002/2014WR016737, 2015.
Wei, Z., Lee, X., Wen, X., and Xiao, W.: Evapotranspiration partitioning for three agro-ecosystems with contrasting moisture conditions: a comparison of an isotope method and a two-source model calculation, Agr. Forest Meteorol., 252, 296–310, https://doi.org/10.1016/j.agrformet.2018.01.019, 2018.
Weller, S., Janz, B., Jörg, L., Kraus, D., Racela, H. S. U., Wassmann, R., Butterbach-Bahl, K., and Kiese, R.: Greenhouse gas emissions and global warming potential of traditional and diversified tropical rice rotation systems, Glob. Change Biol., 22, 432–448, https://doi.org/10.1111/gcb.13099, 2016.
Wenninger, J., Beza, D. T., and Uhlenbrook, S.: Experimental investigations of water fluxes within the soil–vegetation–atmosphere system: Stable isotope mass-balance approach to partition evaporation and transpiration, Phys. Chem. Earth Parts A/B/C, 35, 565–570, https://doi.org/10.1016/j.pce.2010.07.016, 2010.
Zhang, G., Yu, H., Fan, X., Liu, G., Ma, J., and Xu, H.: Effect of rice straw application on stable carbon isotopes, methanogenic pathway, and fraction of CH4 oxidized in a continuously flooded rice field in winter season, Soil Biol. Biochem., 84, 75–82, https://doi.org/10.1016/j.soilbio.2015.02.008, 2015.
Zhou, S., Yu, B., Zhang, Y., Huang, Y., and Wang, G.: Partitioning evapotranspiration based on the concept of underlying water use efficiency, Water Resour. Res., 52, 1160–1175, https://doi.org/10.1002/2015WR017766, 2016.
Zimmermann, U., Münnich, K. O., Roether, W., Kreutz, W., Schubach, K., and Siegel, O.: Tracers Determine Movement of Soil Moisture and Evapotranspiration, Science, 152, 346–347, https://doi.org/10.1126/science.152.3720.346, 1966.
Zwart, S. J. and Bastiaanssen, W. G. M.: Review of measured crop water productivity values for irrigated wheat, rice, cotton and maize, Agr. Water Manage., 69, 115–133, https://doi.org/10.1016/j.agwat.2004.04.007, 2004.