the Creative Commons Attribution 4.0 License.
the Creative Commons Attribution 4.0 License.
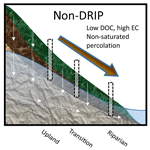
Are dissolved organic carbon concentrations in riparian groundwater linked to hydrological pathways in the boreal forest?
Hjalmar Laudon
Andrés Peralta-Tapia
Lenka Kuglerová
The riparian zone (RZ), or near-stream area, plays a fundamental role in the biogeochemistry of headwaters. Here, wet, carbon-rich soils can change groundwater chemistry before it enters the stream. In the boreal forest, the RZ plays an especially important role in the export of dissolved organic carbon (DOC) to streams. However, the RZ is not uniform, and spatial variability of riparian groundwater hydrology and chemistry can be large. Terrestrial topographic depressions create hydrological pathways towards focal points in the RZ, which we refer to as “discrete riparian inflow points” (DRIPs). Combining the chemical function of the RZ and the convergence of hydrological pathways, we hypothesize that DRIPs play a disproportionally large role in conveying DOC to small streams. Earlier work has demonstrated that runoff from DRIPs can make up the majority of riparian flow contributions to streams, but it is currently unknown how their groundwater chemistry differs from the rest of the RZ. Therefore, we ask the following question: are DOC concentrations in riparian groundwater linked to hydrological pathways in the boreal forest? To answer this question, we sampled riparian groundwater during six campaigns across three boreal headwater streams in Sweden. The groundwater wells were distributed into 10 DRIP and non-DRIP pairs (60 wells), following transects from the upland (20 m lateral distance from the stream bank) to the near-stream area (<5 m lateral distance from the stream bank). The variability in DOC, pH, and electrical conductivity (EC) was analyzed using linear mixed-effects models (LMMs). We explained the variability using three factors: distance from the stream, seasonality, and DRIP/non-DRIP. Our results showed that DRIPs provided DOC-rich water (34 mg L−1) with relatively low EC (36 µS cm−1). The “non-DRIP” riparian water had 40 % lower DOC concentrations (20 mg L−1) and a 45 % higher EC (52 µS cm−1) on average. Moreover, groundwater chemistry from DRIPs was spatially and temporally relatively homogeneous. In contrast, non-DRIP water transformed distinctly in the last 25 m towards the stream, and the chemical variability was also larger between seasons. We concluded that hydrological pathways and spatial variability in riparian groundwater DOC concentrations are linked, and that DRIPs can be seen as important control points in the boreal landscape. Characterizing DRIPs in headwater catchments can be useful for upscaling carbon inputs in boreal stream ecosystems and for delineating hydrologically adapted buffers for forest management practices.
- Article
(3439 KB) - Full-text XML
-
Supplement
(1629 KB) - BibTeX
- EndNote
Headwater streams can be seen as the capillaries of the landscape: although small in appearance, they collectively make up the majority of a stream network. The rich variety in hydrology, biology, and chemistry of headwaters is tightly connected to processes in their catchments (Bishop et al., 2008; Hunsaker and Levine, 1995). Lateral groundwater inputs account for a large part of the streamflow of small streams, magnifying groundwater controls on stream CO2 emissions (Hotchkiss et al., 2015). These controls are governed by groundwater–surface water exchange in the last interface between the landscape and stream ecosystems (Hayashi and Rosenberry, 2002). This near-stream area, the so called riparian zone (RZ), is responsible for important functions such as the chemical transformation of hillslope water (Cirmo and McDonnell, 1997), thermal regulation (Davies-Colley and Rutherford, 2005), and erosion control (Smith, 1976). A few characteristics of the boreal RZ that lead to its unique ecosystem functions are high groundwater levels, a dynamic redox potential, the buildup of soil organic matter, and diverse vegetation (Grabs et al., 2012; Kuglerová et al., 2014b; Lidman et al., 2017). In terms of the hydrological role of the RZ, it has been demonstrated that riparian water dominates streamflow generation, rather than event-based water contributions from hillslopes (McGlynn and McDonnell, 2003). Combined with the chemical transformation of water in the RZ, stream biogeochemistry is therefore largely controlled by RZs (Ledesma et al., 2018b; Lidman et al., 2017). However, RZs are not homogenous strips surrounding surface waters: they contain an array of heterogeneities in hydrogeology, soil development, and vegetation across small spatial scales (Buttle, 2002; Kuglerová et al., 2014b). Moreover, the wetness state changes the chemical function of the RZ in time (Vidon, 2017). Therefore, it is important to further investigate which parts of the RZ matter most for element transport, streamflow generation, and the associated biogeochemical processes.
In hydrological models, streamflow generation has often been conceptualized as a diffuse process, which limits the ability to express points of focused groundwater discharges (Briggs and Hare, 2018). Some models, such as the Riparian Flow-Concentration Integration Model (RIM) and Dominant Source Layer (DSL) concept, have considered the vertical heterogeneity in riparian groundwater fluxes to boreal streams (Ledesma et al., 2015; Seibert et al., 2009). However, it is also necessary to account for hydrological and biogeochemical heterogeneity in riparian groundwater along stream reaches. For example, permanently saturated riparian areas have been identified as the main streamflow generators (Penna et al., 2016) and have been associated with denitrification as well as retention and transformation of (labile) OM compared with drier, oxic, riparian soils (Blackburn et al., 2017; Burgin and Groffman, 2012; Ledesma et al., 2018b). In terms of vegetation, groundwater discharge zones are hotspots for diversity (Kuglerová et al., 2014a). Although these studies show that heterogeneity in the saturation or wetness conditions could be good predictor of heterogeneity in soil chemistry, a connection between spatial variability in groundwater chemistry and hydrological pathways within the riparian–upland continuum has not been demonstrated. The hydrological connection between the upslope catchment, RZs, and the subsequent stream network are highly variable: where some parts of the RZ only drain small individual hillslopes, others function as main hydrological flow paths funneling subsurface water through riparian input zones (Leach et al., 2017). Combining their chemical signature and hydrological upslope connectivity, contributions of such focused riparian inputs could therefore function as important“control points” in the landscape (Bernhardt et al., 2017). The difficulty is that incorporating these control points into models or practical applications means that they have to be characterized in order to explain stream dynamics. Especially for informing distributed models that surpass the catchment scale, the determination and characterization of these control points remains one of the challenges for the scientific community (Briggs and Hare, 2018).
For the hydrological characterization of riparian inputs, various approaches can be used across scales. Although subsurface pathways do not entirely follow surface topography (Devito et al., 2005), it has been demonstrated that topographic depressions are a good indicator of accumulation areas of water, ponding, shallow groundwater tables, and concentrated flow paths in the near-stream area (Ågren et al., 2014; Jencso et al., 2009; Wallace et al., 2018). As such, topographic models can predict where along a stream network disproportionally large amounts of groundwater connect with the stream. Mixing models using water temperature and chemistry can further depict whether the topography-based predictions of focused riparian inputs to streams are in line with reality (Leach et al., 2017). These discrete riparian inflow points (DRIPs; Fig. S2), provide continuous flows of subsurface water during low-flow periods, but they have also been observed to be highly dynamic in their activation during hydrological events (Ploum et al., 2018). Contrary to the incorporation of water from ephemeral streams in perennial stream networks or the connection of intermittent sections of a stream network (Ågren et al., 2015), DRIPs are dominated by subsurface flowing water, and the discharge to the stream is the first exposure to an open channel. A recent study demonstrated the temporal dynamics in increasing greenhouse gas evasion from the stream reach in close downstream proximity of DRIPs (Lupon et al., 2019). Moreover, in Arctic systems the presence of riparian wet areas has partially explained stream CO2 evasion (Rocher-Ros et al., 2019). The latter suggests that both the hydrological fluxes and the biogeochemical reactions in the stream are associated with the hydrological activity of DRIPs. However, in order to determine whether DRIPs matter for stream biogeochemistry, chemical characterization of the discharging groundwater is needed.
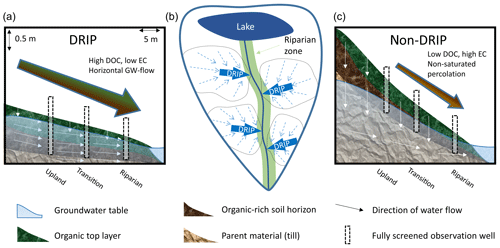
Figure 1Conceptual illustration of the two types of hypothesized riparian areas along a boreal stream. (b) Discrete riparian inflow points (DRIPs) are focal points in the riparian zone (light green) where pathways converge before reaching the stream. DRIPs typically have flatter topography and higher groundwater tables than non-DRIPs. Panels (a) and (c) show DRIP and non-DRIP riparian zones, respectively. Green layers represent the approximate extent of the organic layer, brown layers are riparian soils with high organic matter content, light brown layers represent parent material, transparent blue overlay represents the groundwater table, and black bars represent well transects of DRIP areas in panel (a) and non-DRIP areas in panel (c). Large arrows suggest the relative hydrological contribution, and the color fill of the arrows matches the soil layer with which the groundwater interacted most.
Characterizing groundwater chemistry is an especially challenging task. Previously this challenge has been bypassed by inferring groundwater chemistry from the baseflow chemistry of streams (Peralta-Tapia et al., 2015; Tetzlaff and Soulsby, 2008). Furthermore, the RIM model has provided a framework to infer groundwater chemistry profiles from stream chemistry (Seibert et al., 2009). However, even at the local scale, spatial variability in groundwater chemistry overrules temporal variation and requires regular sampling of extensive well networks (Kiewiet et al., 2019). Within meters of each other, groundwater signatures can vary greatly (Penna et al., 2016). Three key parameters for the chemical characterization of groundwater in boreal forests are dissolved organic carbon (DOC), pH, and ionic strength. DOC concentrations in groundwater are the result of the interaction between water and carbon-rich materials in the shallow subsurface environment that are associated with paludification (Lavoie et al., 2005). More specifically, for near-stream areas, the width of the RZ is associated with the size of the potential carbon pool and the subsequent DOC concentrations (Ledesma et al., 2015). Apart from its role in food web structures and carbon transport, DOC also increases the acidity (decreases the pH) of soils and surface waters (Buffam et al., 2007). Electrical conductivity (EC) can be used as a proxy for the ionic strength, or the total amount of dissolved ions in water (Corwin and Lesch, 2005). Water contact time with minerals and weathering processes are important factors determining EC (Saarenketo, 1998), with increasing EC indicating longer interactions (Hayashi, 2004; Peralta-Tapia et al., 2015).
In the context of the spatial variability of riparian groundwater chemistry, it can be expected that DOC, pH, and EC differ between DRIP and non-DRIP riparian areas (Fig. 1). DRIPs are associated with high groundwater levels and wet, organic-rich soils with vegetation that favors wet conditions, whereas non-DRIPs have drier top soils and deeper groundwater levels (Kuglerová et al., 2014a). Inherent to their topographic setting, DRIPs drain a large upland area, whereas non-DRIPs typically drain only a small surrounding area of the RZ or they are recharge zones for adjacent DRIPs. Moreover, the water in DRIPs travels a longer distance horizontally in what is presumably wet, highly permeable, organic-rich soil. Non-DRIP water, in contrast, is likely to infiltrate vertically through an oxic, organic-rich top soil, before being transported a relative short distance horizontally through supposedly more mineral substrate. This implies that the contact time of the water with wet, organic soil and drier, mineral soil is different in both cases, which should lead to contrasting water chemistry.
In this study, we characterize groundwater in a paired well network that is specifically designed to incorporate (saturated) riparian areas with large contributing areas (DRIPs) and drier parts of the RZ with small contributing areas (non-DRIPs). We hypothesize that groundwater in DRIPs has higher DOC concentrations and lower pH than non-DRIPs. The deeper groundwater levels in non-DRIP areas and the longer contact times with mineral soil relative to organic soil leads us to expect that EC will be higher in non-DRIP water compared with DRIPs. Furthermore, we discuss the implications of using a binary categorization of the RZ opposed to continuous, process-based approaches.
To test our hypothesis we collected DRIP and non-DRIP groundwater across a riparian gradient during different seasons. Using linear mixed-effects models (LMMs), we analyzed the role of DRIPs on the biogeochemical composition of riparian groundwater in relation to spatial and temporal variability. We performed our study in Krycklan, a boreal forested catchment in northern Sweden.
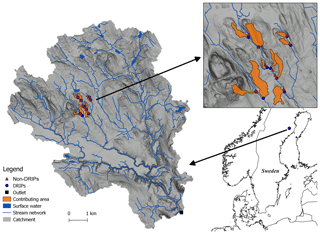
Figure 2Krycklan catchment in northern Sweden. The upper right panel shows the particular study area where the well network was installed. The red triangles and blue dots indicate non-DRIP and DRIP transects, respectively, which consist of three wells placed at 20, 10, and <5 m from the stream. The black square indicates the catchment outlet. The contributing areas of each well transect are indicated in orange. Non-DRIP contributing areas are typically too small to be depicted.
2.1 Study area
The Krycklan catchment is situated near the town of Vindeln, Sweden (64∘14′ N, 19∘46′ E; Fig. 2). The bedrock is predominately Svecofennian metasediments and metagreywacke. Quaternary deposits consist mostly of till (51 %) and sorted sediments (30 %). Land cover is dominated by forest (87 %), and there is 9 % mire cover. Furthermore, there are sporadically thin soils and bedrock as well as a small fraction of arable land (2 %). The climate is characterized as cold humid temperate type, with almost 6 months of snow cover. The yearly average temperature is 1.8 ∘C, annual precipitation is 614 mm, and the annual mean runoff approximates 311 mm (Laudon et al., 2013). The well network is situated along streams referred to as C4, C6, and C8 (Laudon et al., 2013), with a drainage area of 18, 110, and 230 ha, respectively. Catchments C4 and C6 have been widely studied with respect to lateral flow and groundwater and surface water interaction, and the catchments may be referred to as Kallkälsmyrsbäcken and Stortjärnsbäcken in other studies (Laudon et al., 2004b, 2007). At the C6 hydrological stations, flows vary from a few liters per second baseflow to 200 L s−1 peak flows (Ploum et al., 2018). The yearly hydrograph is characterized by sustained baseflow throughout the winter months, followed by spring snowmelt floods in April and May (Fig. S1 in the Supplement). In summer and fall low-flow conditions are common with occasional rain-induced flow events. From November onward, flow reduces as temperatures fall below 0 ∘C and baseflow conditions set in.
2.2 Site selection and sampling well infrastructure
Discrete riparian inflow points (DRIPs) were selected by considering wet areas, based on a topographic wetness index, and selecting large step changes in the catchment area along stream networks using flow accumulation algorithms (Ågren et al., 2014; Beven and Kirkby, 1979; O'Callaghan and Mark, 1984). DRIPs were typically recognized in the field as wet corridors in the forest characterized by their flat topography, wet soil conditions, moss-dominated vegetation, and decrease in tree density (Fig. S2). The DRIPs (n = 10) were selected with contributing upslope area varying from 0.6 to 7.7 ha, with a mean contributing area of 2.7 ha. Non-DRIPs had an upslope contributing area between 4 and 80 m2 (on average 17 m2). The DRIPs have been field-validated (Ploum et al., 2018) and surveyed with respect to species richness (Kuglerová et al., 2014a). For some sites, chemical and thermal signatures further corroborated the location where riparian water discharged into the stream (Leach et al., 2017).
The setup of this study consists of a well network with a total of 60 fully screened PVC wells (30 mm diameter) arranged in 10 paired transects. Each transect consisted of a riparian well, which was typically situated between 1 and 5 m from the stream; a transition well, which was approximately 10 m from the stream; and an upland well, which was 20 m from the stream. Transects followed the local topography in order to approximate local hydraulic gradients and flow paths. The non-DRIP transects were installed close (<50 m) to each DRIP transect to ensure similarity in local conditions. All wells were drilled until resistance or until an aquitard layer was reached. Riparian wells had a mean depth of 95 cm (σ=37 cm), transition wells had a mean depth of 99 cm (σ=42 cm), and upland wells had a mean depth of 121 cm (σ=55 cm). We assumed that the water sampled from the well was a weighted average of the phreatic aquifer, down to the depth of the well. Given the exponentially decaying hydraulic conductivity with depth, this assumption would imply that, under fully saturated conditions, the majority of the water is from the upper soil layers, referred to as the dominant source layer (Ledesma et al., 2015). Given that context, lateral flow below the well bottom was considered negligible compared with the flow in the vertical domain of our well installations. For a small subset of riparian sampling wells, water levels were available from directly neighboring wells (<2 m apart). Figure S1 shows an exemplar time series of these wells and a hydrograph for 2018. The mean depth to the water table for those time series was 9.6 cm (σ=4.2 cm) for DRIP wells and 54.5 cm (σ=17.3 cm) for non-DRIP wells.
2.3 Groundwater sampling and chemical analysis
The well network was sampled using suction cup lysimeters and vacuumed glass bottles (Blackburn et al., 2017). The wells were pumped before the suction cups were installed to ensure that water from the aquifer was sampled without any stagnant well water. The bottles were collected after approximately 24 h and subsampled, filtered, and analyzed within 48 h. In addition, a more intensive sampling campaign was conducted for a series of riparian wells only. These were sampled following a similar protocol, but instead of suction cup lysimeters, a peristaltic pump was used for the collection of water samples.
Water samples were collected during spring, summer, and fall of the hydrological years 2016 (Q=328 mm, P=629 mm) and 2017 (Q=259 mm, P=572 mm). In total, 359 samples were analyzed from six sampling campaigns, of which 200 were from DRIP wells and 159 were from non-DRIP wells. Non-DRIP wells occasionally had water levels that were too low to collect a representative water sample. For the analysis of dissolved organic carbon (DOC), a subsample was filtered (0.45 µm) into acid-washed high-density polyethylene bottles (rinsed three times) and kept at 4 ∘C before laboratory analysis. DOC was measured by acidifying the sample and subsequently combusting it using a Shimadzu TOC-VCPH. The pH and EC were subsampled without headspace into acid-washed high-density polyethylene bottles (rinsed three times) and kept at 4 ∘C before laboratory analysis. Samples were analyzed using a METTLER TOLEDO DGi117-Water probe for pH and a METTLER TOLEDO InLab741 probe for EC.
2.4 Statistical analysis
We used linear mixed-effects models (LMMs) to analyze patterns in DOC, pH, and EC. The analysis was performed in R using “lmer” models from the “lme4” R package (Bates and Maechler, 2009; Bates et al., 2014). The LMMs provided a nonparametric approach to explain variability in the response variables due to fixed effects (factors that were included in the study design) and random effects. Random effects account for factors that were not part of the study design but possibly affected variability in DOC, pH, and EC. The fixed effects considered in this study were the hydrological pathways (HP; DRIP and non-DRIP), the position in the landscape relative to the stream (POS; riparian, transition, and upland), the season when the samples were taken (TIME; spring, summer, and fall), and the two-way interaction of HP with POS and TIME, respectively. The random effects included were the stream identity and the transect identity along which the wells were situated. Thus, we accounted for specific catchment and hillslope properties. The model structure selection was based on the lowest AIC (Akaike information criterion).
We evaluated the model performance using Type II Wald F tests with Kenward–Roger degrees of freedom (as all explanatory variables are factors). F statistics indicate the explained variance as a ratio of the unexplained variance. An effect was considered significant if p values < 0.05. We evaluated the assumption of a Gaussian distribution of errors by inspecting residuals and quantile distributions. For DOC and pH, five outliers and two outliers were removed from the upper quantile, respectively. For EC, one outlier was removed from the lowest tail and two outliers were removed from the highest tail of the distribution. For comparing the contrasts of levels within explanatory factors (for example DRIP vs. non-DRIP comparisons), we investigated least square means using the “lsmeans” R package, including a Tukey adjustment to account for potential differences in sample size (Lenth et al., 2016). Furthermore, the marginal and conditional coefficients of determination ( and ) were presented to compare the explained variance due to the fixed effects as well as the variance explained by the fixed and random effects combined (“MuMln” R package, Barton, 2014).
3.1 DOC
The water collected in wells situated in the DRIPs had a higher mean DOC concentration (33.9 mg L−1) than non-DRIP wells (19.9 mg L−1, Fig. 3). DOC concentrations in DRIPs increased from upland wells (29.2 mg L−1) towards the riparian wells (36.3 mg L−1), whereas in non-DRIP riparian wells, the DOC concentration increased from 16.4 to 20.1 mg L−1 (DF = 19, p=0.03). When we only accounted for gradients between upland and riparian wells (without distinction between DRIP or non-DRIP sites), the differences were not as large, but they were still significant (from 22.8 to 28.2 mg L−1, DF = 327, p=0.0001). Although DOC concentrations in the upland groundwater of DRIPs was already high, the overall gain in DOC concentrations from the upland to the riparian wells was mostly attributed to DRIPs (DF = 326, p=0.0003). Average DOC concentrations were contrasting in the upland wells (29.2 and 16.4 mg L−1 for DRIP and non-DRIPs, respectively) but were statistically not significant (p=0.1844; Fig. 4, upper left panel). In summer and fall, DOC concentrations in DRIP groundwater (36.4 and 33.3 mg L−1) were twice as high as in non-DRIP groundwater (18.0 and 17.7 mg L−1; Fig. 5, upper panels). However, during snowmelt in spring, this difference decreased. This change was the result of an of average 20 % decrease in DOC concentrations in DRIPs (28.5 mg L−1) compared with the summer average. In non-DRIP areas, there were no significant contrasts, although there was a small increase in spring (21.6 mg L−1) compared with summer and fall (18.0 and 17.7 mg L−1, p=0.4986 and p=0.3019, respectively). Overall, the fixed effects alone explained 22 % of the variance in the DOC found in the groundwater well network. With the random effects included, the explained variance was 68 %.
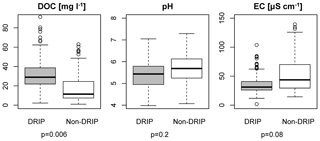
Figure 3Groundwater chemistry of DRIPs vs. non-DRIPs. DRIP boxplots are presented in gray, and non-DRIP boxplots are shown in white. Each panel represents one response variable. Whiskers represent the 25th and 75th percentile. The p values were obtained using an F test, and p values < 0.05 were considered significant.
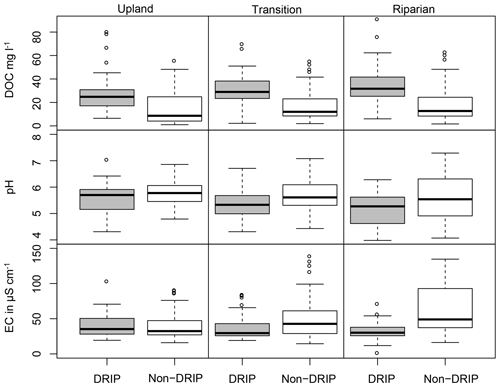
Figure 4Groundwater chemistry gradients from upland to riparian wells. In each column DOC, pH, and EC values are presented for a location relative to the stream (riparian, transition, and upland). Within each panel, DRIP boxplots are presented in gray, and non-DRIP boxplots are shown in white.
3.2 pH
Although typically associated with DOC, the pH was not as distinctly different between DRIP and non-DRIP water as DOC (Fig. 3). Overall, the fixed effects accounted for 13 % of the variance, whereas 55 % of the variance was accounted for when including random effects (Table 1). Mean pH levels were 5.38 for DRIPs and 5.66 for non-DRIPs (DF = 16, p=0.2). The position in the landscape had more effect on the variability in pH: the upland pH was similar at DRIPs and non-DRIPs and decreased towards the riparian area (5.66 to 5.40, p<0.0001). Although no significant effect was found with respect to the interaction between the landscape position and hydrological conditions (Table 1), the least square means analysis showed a pronounced decrease in pH from upland to riparian wells in the DRIP areas (5.57 to 5.19, p<0.0001; Fig. 4, middle row). The second important explanatory variable was seasonality (TIME in Table 1). The most notable was the increasing pH from summer to fall (5.37 to 5.70, p<0.0001) in both DRIP and non-DRIP areas (Fig. 5, the center and right panel in the middle row). In the transition to spring, the pH decreased again (pHspring=5.48), mostly due to a shift in the DRIPs (p=0.04). Furthermore, the variability in the pH in non-DRIP water was high compared with DRIP areas, especially during summer (Fig. 5, center panel).
Table 1Summary of statistics from the LMM models for DOC, pH, and EC. The three columns show the response variables DOC, pH, and EC. The upper two rows show the marginal and conditional coefficients of determination ( and ), which explain the variance due to the fixed effects as well as the variance due to the fixed and random effects combined. For each explanatory variable and the interaction with hydrological pathways (HP), the p value and F statistic are presented. HP differentiates between DRIPs and non-DRIPs. POS represents the three positions in the along-transect areas: riparian, transition, and upland. TIME represents the three different seasons in which sampling took place: spring, summer, and fall. Significance is defined as follows: p<0.001 “”, “”, “*”, “.”, and p>0.1 “–”. Explanatory variables displayed as “variable1:variable2” represent the interaction between both variables.
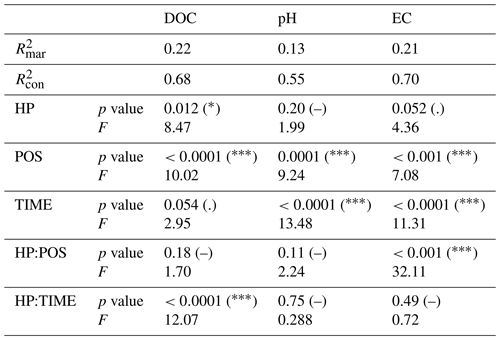
3.3 EC
Mean electrical conductivity from DRIP water was 36.2 µS cm−1, which was lower (p=0.08) than the mean of non-DRIP water (51.6 µS cm−1, Fig. 3). The variance in EC was mostly explained by POS and TIME as well as the interaction between HP and POS (Table 1). Overall, the conductivity increased from the upland to the riparian wells (39.3 to 48.0 µS cm−1) and also increased from spring to fall (39.7 to 48.7 µS cm−1; Fig. 4, lower panels). The interactions between groundwater conditions and the position relative to the stream were mostly related to two specific contrasts. The variability in EC in non-DRIP groundwater increased from the upland to riparian wells, whereas the EC remained stable in DRIP areas (Fig. 4, bottom row). Moreover, large differences were found between DRIPs and non-DRIPs in the riparian wells, where the EC in non-DRIP riparian areas was twice as high as the EC in DRIPs (63.6 µS cm−1 compared with 32.4 µS cm−1). In the upland areas, the DRIP and non-DRIP water was similar. Non-DRIP water increased from 40.5 to 62.4 µS cm−1 from the upland wells towards the riparian wells, whereas DRIPs even decreased with respect to conductivity (38.2 and 32.4 µS cm−1 for upland and riparian wells). Over the different seasons (TIME), the contrasts between DRIP and non-DRIP chemistry were consistent (Fig. 5, lower panels). The interaction between groundwater and seasonality (TIME) was not found to have an effect on EC. The only specific contrasts for both DRIP and non-DRIP was a 5 µS cm−1 decrease from fall to spring (PDRIP=0.05, Pnon-DRIP=0.0007). Overall, the explained variance of our LMM was 70 % for EC compared with 22 % when only fixed effects were accounted for (Table 1).
Our riparian groundwater sampling campaigns demonstrated that DOC concentrations in DRIPs were almost twice as high as less hydrologically active riparian areas (non-DRIPs) on average. Groundwater chemistry of DRIPs was more constant from the upland to the RZ, and it remained relatively stable across seasons compared with non-DRIPs. The groundwater chemistry of non-DRIPs was characterized by 40 % higher EC values than DRIPs as well as increasing variability towards the stream and across seasons. Differences in pH were less distinct and were mostly attributed to seasonal changes. These results confirm our hypothesis that DRIPs have a more DOC-rich groundwater chemistry associated with organic soils, whereas non-DRIP water can be associated with chemistry originating from mineral-soil-dominated systems. However, apart from the commonly tested factors, we found that site-specific properties play a major role in explaining the spatiotemporal variability in the chemistry of groundwater.
These findings demonstrated that DRIPs and non-DRIPs appear to be dominated by different processes. DRIPs already have a distinct DOC-rich groundwater chemistry upland of the near-stream area, high groundwater tables, and typically flat local topographic gradients. They could be considered to be cryptic wetlands (Creed et al., 2003), with the exceptional property of linking a large upland area to the RZ and, subsequently, the stream network. Contrary to this, the non-DRIP transects were characterized by distinct increasing EC, deeper and more fluctuating groundwater tables (Fig. S1), and steeper local topography. These transects resembled typical riparian hillslopes with vertical chemistry profiles that have been studied intensively in this study area (e.g., Grabs et al., 2012; Ledesma et al., 2013; Lidman et al., 2017). As such, the large spatial variability in non-DRIP groundwater chemistry supports the idea of dynamic water tables across the RZ that drive chemical variability via the activation of different soil layers, such as the DSL. Our results reflected this through the large temporal variability in non-DRIP groundwater chemistry across seasons. Thus, riparian groundwater inputs from non-DRIPs are likely to be most relevant during hydrological events (when the groundwater table becomes increasingly dynamic), whereas DRIPs provide groundwater inputs during both hydrological events and low-flow periods.
Our results showed that a significant difference can also be found between upland and riparian groundwater in DOC, pH, and EC without the DRIP/non-DRIP distinction (Table 1, POS). From upland to riparian wells, groundwater was enriched in DOC and EC. This demonstrated that existing 2-D conceptual models of chemical enrichment across riparian hillslope apply to our well network as well (Ledesma et al., 2018a). However, from a longitudinal point of view along the stream, our distinction of DRIP and non-DRIP transects allowed us to further highlight DRIPs as specific areas of interest across the RZ–stream interface for groundwater inputs. For seasons (TIME), we also observed significant differences between spring, summer, and fall for pH and EC as well as a close to significant difference for DOC (Table 1, TIME). The sampling campaigns represent seasonal snapshots that mostly demonstrate a higher pH and decreased EC in fall, whereas summer and spring samplings were, without the distinction of DRIPs and non-DRIPs, similar with respect to pH and EC. However, when we accounted for DRIP and non-DRIP transects, more processed-based interpretations could be made. Specifically, we found that the high DOC concentrations in DRIP groundwater decreased by 20 %, and became less spatially variable during spring flood conditions. We believe that the snowmelt dilution of groundwater is a likely cause of the decreased DOC concentrations during spring, given that the fully screened wells represent groundwater from the entire vertical soil profile, including overland (or over-ice) flow. Furthermore, ice sheet formation in the DRIP areas has previously been reported, which can route water over the ice surface instead of the organic-rich subsurface flow paths, such as the DSL (Ploum et al., 2018). These overland-flow findings are similar to the dilution effects and soil frost effects reported for wetland-dominated streams during spring floods (Laudon et al., 2004a, 2011). In contrast to DRIPs, riparian groundwater in non-DRIP areas increased in DOC and in variability during the snowmelt season (from 17.7 to 21.6 mg L−1). This is likely associated with the increase in the groundwater level (Fig. S1), and the activation of the dominant source layer in the upper section of the soil (Ledesma et al., 2015). The increased variability could also be related to different timing of rising groundwater levels, for example due to local conditions that affect snow melt rates on hillslopes such as shading or sun exposure. As such, our sampling campaigns provided a snapshot of the elapse of the snowmelt flood.
With the comparison of riparian groundwater chemistry using the DRIP/non-DRIP concept, we have studied two different riparian hydrological connectivity types: DRIPs had hydrological connection with large upslope contributing areas (2.7 ha on average) as well as mostly saturated soil conditions (Fig. S1), whereas non-DRIPs were characterized by draining individual hillslopes (17 m2 on average) and having lower groundwater levels in the RZ (Fig. S1). Earlier work in the study area has demonstrated that the extent of the RZ and contributing area play an important role in the available soil carbon pool and the related DOC export from RZs to streams (Ledesma et al., 2015). However, the latter covers RZs with contributing areas that range between 2.5 and 1500 m2. Between such riparian hillslope contributing areas and the initiation of streams (e.g., 10–20 ha), there is a wide range of features that focus water towards the perennial network. Where ephemeral streams are often a clear extension of the stream channel, which activate mostly during hydrological events (Ågren et al., 2015), DRIPs have no such stream-like features and should be more associated with the terrestrial landscape than the stream network. Such features have been represented in different landscapes across the world and highlight specific processes such as the groundwater discharge zone, groundwater hotspots, cryptic wetlands, swales, focused seepage, discrete seepage, springs, upwelling zones, preferential discharge, and zero-order basins (Creed et al., 2003; Hayashi and Rosenberry, 2002; Tsuboyama et al., 2000). By using the term DRIPs, we aimed to fill the gap between riparian hillslopes and (fractal) stream networks as riparian landscape features that have hydrological connections to large upland contributing areas but lack stream channel formation.
Given the stable DOC concentrations and the large role in streamflow generation (Leach et al., 2017; Ploum et al., 2018), the DRIP concept could potentially be used to scale riparian contributions to headwaters at the catchment level (Laudon and Sponseller, 2018). A preliminary analysis showed that 57 % of the Krycklan catchment drains into the stream network through DRIPs, which spatially cover only 12 % of the RZ (the preliminary analysis is given in the Supplement). However, the topography-driven approach behind our DRIP concept might miss certain contributions that are not necessarily related to surface topography, especially in areas where phreatic aquifers are not underlain by till deposits, or on scales that surpass the headwater basins (Devito et al., 2005). Previous work has demonstrated that the input of deeper/older groundwater (with high EC) increases with drainage area in boreal catchments, up to a threshold where old and new groundwater input reach a balance (Peralta-Tapia et al., 2015). Future work could be directed towards the further chemical analysis of DRIPs and non-DRIPs and their role in groundwater–surface water interactions throughout the catchment. Although the visible effect of DRIPs on streams likely decreases in higher-order streams, links between hydrological pathways and groundwater chemistry dynamics have been found to significantly affect the chemistry of a fifth-order river (Carlyle and Hill, 2001). Further, flow paths known as “water tracks” have been shown to be important biogeochemical controls on higher-order Arctic rivers (Harms and Ludwig, 2016; McNamara et al., 1999).
Spatial characterization of groundwater chemistry has been studied as an integrated signal of the phreatic aquifer (Kiewiet et al., 2019), as well as using piezometers or lysimeters at specific depths, to depict vertical chemical profiles (Grabs et al., 2012; Lidman et al., 2017). Our approach was considered to represent a mixture of riparian groundwater that is likely to flow into the stream during various hydrological conditions. Where the aforementioned studies relate vertical water chemistry profiles to water level fluctuations to obtain process-based understanding, our study focused on finding patterns in generalizable factors such as spatial distributions (upland to riparian), different seasons (spring, summer, and fall), and hydrological connectivity. Thus, our study can be contextualized as an approach that potentially allows for the characterization of control points in the landscape with the use of minimal information. The relative contributions and biogeochemical characteristics of DRIP and non-DRIP RZs in the longitudinal dimension can potentially be combined with models that specify vertical profiles of groundwater chemistry, such as the RIM model (Seibert et al., 2009). As such, we can identify which areas within the RZ exert a large control on stream water quality and quantity.
Along the stream networks, the delineation of DRIP/non-DRIP areas in the RZ can help to implement hydrologically adapted buffers in forest management, thereby ensuring that water bodies maintain a good water quality (Kuglerová et al., 2014b; Tiwari et al., 2016; Wallace et al., 2018). Traditionally, forest practices considered fixed width buffers, even though the riparian function is not homogeneous around all water bodies (Buttle, 2002). Besides the extent of the riparian soils (Ledesma et al., 2018b), species richness within the RZ (Kuglerová et al., 2014a), and the extent of (ephemeral) stream networks (Ågren et al., 2015), our results support that variable widths should be considered in riparian buffer management. We found that DRIP water already had a distinct chemical signature before entering the RZ: 80 % of the DOC originated from upland riparian wells. This suggests that the chemical role that is associated with RZs extends further away from the stream than traditional fixed-width buffer management considers.
For the identification of control points, improving hydrological models, and sustainable forest management practices, a binary approach with little need for local properties can be a very useful tool. However, to understand the underlying mechanisms and the link to the landscape, the hydrology of RZs should be considered nonbinary (Klaus and Jackson, 2018). Our LMMs showed that a large part of the variance is explained by the random effects, which contain information regarding the unique properties of individual transects and to a lesser extent the subcatchments. The large variation in non-DRIPs leads to statistically weak contrasts, but this does not mean non-DRIP RZs are less important. We demonstrated that important chemical changes also occur in riparian non-DRIP areas, but their complexity surpassed the binary simplifications made in this study design. To explain the variance that was accounted for using random factors, it could be of interest to further analyze local landscape characteristics, subsurface soil properties, and groundwater level dynamics to decipher whether soil, biology, or hydrology define biochemical characteristics throughout the RZ.
Are DOC concentrations in riparian groundwater linked to hydrological pathways in the boreal forest? Yes, based on our findings there is a strong link between the hydrological pathways in the RZ and the DOC concentrations of riparian groundwater. At the confluence of hydrological pathways in the RZ (discrete riparian inflow points – DRIPs), we found groundwater with an organic-rich, relatively stable chemistry, compared with the remaining, drier riparian areas. Importantly, DRIPs seem to be supplying this chemically distinct groundwater independently of time and space. Combining the organic-rich chemical characteristic and dominant hydrological contributions to headwaters, DRIPs fulfill a specific role in explaining longitudinal variability of DOC concentrations along stream reaches. We propose that DRIPs can be control points in the boreal riparian forest for the transport of carbon to small streams. To our knowledge, this study is the first to characterize spatial groundwater chemistry that incorporated hydrological pathways in the study design a priori.
However, to fully evaluate the impact of DRIPs on stream water generation and the associated stream chemistry, there is the need to further investigate the hydrological activation and a broader chemical characterization. To understand the mechanisms and processes that link hydrological pathways and groundwater chemistry in boreal forest, we suggest to focus on nonbinary approaches incorporating groundwater fluctuations, soil properties, and landscape characteristics.
Data are available upon reasonable request from the first author (stefan.ploum@slu.se). Krycklan data are openly available from the Svartberget database: https://franklin.vfp.slu.se/ (last access: April 2020).
The supplement related to this article is available online at: https://doi.org/10.5194/hess-24-1709-2020-supplement.
LK and HL conceptualized the study design and methodology, and supervised the data analysis, interpretation, and writing processes. APT conducted the well installation, provided field support, reviewed the written text, and was involved in discussions. SWP was responsible for the collection of data and the data analysis, and the figures, interpretation, and writing and revision of the paper.
Hjalmar Laudon is a member of the editorial board of this special issue of Hydrology and Earth System Sciences.
This article is part of the special issue “Linking landscape organisation and hydrological functioning: from hypotheses and observations to concepts, models and understanding (HESS/ESSD inter-journal SI)”. It is not associated with a conference.
We acknowledge Anna Lupon and the staff at the Svartberget Research Station for field support. We thank Jason Leach and Ilja van Meerveld for contributing to the discussions and hydrological interpretations.
This research has been supported by the Oscar and Lili Lamm Foundation and the Swedish Research Council Formas (grant no. 2015-00531). The Krycklan catchment is also supported by SITES (VR), SKB, KAW, and the Kempe Foundation.
This paper was edited by Loes van Schaik and reviewed by two anonymous referees.
Ågren, A. M., Lidberg, W., Strömgren, M., Ogilvie, J., and Arp, P. A.: Evaluating digital terrain indices for soil wetness mapping – a Swedish case study, Hydrol. Earth Syst. Sci., 18, 3623–3634, https://doi.org/10.5194/hess-18-3623-2014, 2014.
Ågren, A., Lidberg, W., and Ring, E.: Mapping temporal dynamics in a forest stream network – implications for riparian forest management, Forests, 6, 2982–3001, 2015.
Barton, K.: Multi-model inference, R package MuMIn version 1.10.5:46, available at: https://cran.r-project.org/web/packages/MuMIn/ (last access: April 2020), 2014.
Bates, D. M. and Maechler, M.: lmer4: linear mixed-effects models using S4 classes, R package version 1.1-12, available at: https://cran.r-project.org/web/packages/lme4/ (last access: April 2020), 2009.
Bates, D., Mächler, M., Bolker, B., and Walker, S.: Fitting linear mixed-effects models using lme4, arXiv preprint, arXiv:1406.5823, 2014.
Bernhardt, E. S., Blaszczak, J. R., Ficken, C. D., Fork, M. L., Kaiser, K. E., and Seybold, E. C.: Control Points in Ecosystems: Moving Beyond the Hot Spot Hot Moment Concept, Ecosystems, 20, 665–682, 2017.
Beven, K. J. and Kirkby, M. J.: A physically based, variable contributing area model of basin hydrology/Un modèle à base physique de zone d'appel variable de l'hydrologie du bassin versant, Hydrolog. Sci. J., 24, 43–69, 1979.
Bishop, K., Buffam, I., Erlandsson, M., Fölster, J., Laudon, H., Seibert, J., and Temnerud, J.: Aqua Incognita: the unknown headwaters, Hydrol. Process., 22, 1239–1242, 2008.
Blackburn, M., Ledesma, J. L., Näsholm, T., Laudon, H., and Sponseller, R. A.: Evaluating hillslope and riparian contributions to dissolved nitrogen (N) export from a boreal forest catchment, J. Geophys. Res.-Biogeo., 122, 324–339, 2017.
Briggs, M. A. and Hare, D. K.: Explicit consideration of preferential groundwater discharges as surface water ecosystem control points, Hydrol. Process., 32, 2435–2440, 2018.
Buffam, I., Laudon, H., Temnerud, J., Mörth, C.-M., and Bishop, K.: Landscape-scale variability of acidity and dissolved organic carbon during spring flood in a boreal stream network, J. Geophys. Res.-Biogeo., 112, G01022, https://doi.org/10.1029/2006JG000218, 2007.
Burgin, A. J. and Groffman, P. M.: Soil O2 controls denitrification rates and N2O yield in a riparian wetland, J. Geophys. Res.-Biogeo., 117, G01010, https://doi.org/10.1029/2011JG001799, 2012.
Buttle, J.: Rethinking the donut: the case for hydrologically relevant buffer zones, Hydrol. Process., 16, 3093–3096, 2002.
Carlyle, G. and Hill, A.: Groundwater phosphate dynamics in a river riparian zone: effects of hydrologic flowpaths, lithology and redox chemistry, J. Hydrol., 247, 151–168, 2001.
Cirmo, C. P. and McDonnell, J. J.: Linking the hydrologic and biogeochemical controls of nitrogen transport in near-stream zones of temperate-forested catchments: a review, J. Hydrol., 199, 88–120, 1997.
Corwin, D. and Lesch, S.: Apparent soil electrical conductivity measurements in agriculture, Comput. Eelectron. Agricult., 46, 11–43, 2005.
Creed, I., Sanford, S., Beall, F., Molot, L., and Dillon, P.: Cryptic wetlands: integrating hidden wetlands in regression models of the export of dissolved organic carbon from forested landscapes, Hydrol. Process., 17, 3629–3648, 2003.
Davies-Colley, R. J. and Rutherford, J. C.: Some approaches for measuring and modelling riparian shade, Ecol. Eng., 24, 525–530, 2005.
Devito, K., Creed, I., Gan, T., Mendoza, C., Petrone, R., Silins, U., and Smerdon, B.: A framework for broad-scale classification of hydrologic response units on the Boreal Plain: is topography the last thing to consider?, Hydrol. Process., 19, 1705–1714, 2005.
Grabs, T., Bishop, K., Laudon, H., Lyon, S. W., and Seibert, J.: Riparian zone hydrology and soil water total organic carbon (TOC): implications for spatial variability and upscaling of lateral riparian TOC exports, Biogeosciences, 9, 3901–3916, https://doi.org/10.5194/bg-9-3901-2012, 2012.
Harms, T. K. and Ludwig, S. M.: Retention and removal of nitrogen and phosphorus in saturated soils of arctic hillslopes, Biogeochemistry, 127, 291–304, 2016.
Hayashi, M.: Temperature-electrical conductivity relation of water for environmental monitoring and geophysical data inversion, Environ. Monit. Assess., 96, 119–128, 2004.
Hayashi, M. and Rosenberry, D. O.: Effects of ground water exchange on the hydrology and ecology of surface water, Groundwater, 40, 309–316, 2002.
Hotchkiss, E., Hall Jr., R., Sponseller, R., Butman, D., Klaminder, J., Laudon, H., Rosvall, M., and Karlsson, J.: Sources of and processes controlling CO2 emissions change with the size of streams and rivers, Nat. Geosci., 8, 696–699, 2015.
Hunsaker, C. T. and Levine, D. A.: Hierarchical approaches to the study of water quality in rivers, BioScience, 45, 193–203, 1995.
Jencso, K. G., McGlynn, B. L., Gooseff, M. N., Wondzell, S. M., Bencala, K. E., and Marshall, L. A.: Hydrologic connectivity between landscapes and streams: Transferring reach-and plot-scale understanding to the catchment scale, Water Resour. Res., 45, W04428, https://doi.org/10.1029/2008WR007225, 2009.
Kiewiet, L., von Freyberg, J., and van Meerveld, H.: Spatiotemporal variability in hydrochemistry of shallow groundwater in a small pre-alpine catchment: the importance of landscape elements, Hydrol. Process., 33.19, 2502–2522, https://doi.org/10.1002/hyp.13517, 2019.
Klaus, J. and Jackson, C. R.: Interflow Is Not Binary: A Continuous Shallow Perched Layer Does Not Imply Continuous Connectivity, Water Resour. Res., 54, 5921–5932, 2018.
Kuglerová, L., Jansson, R., Ågren, A., Laudon, H., and Malm-Renöfält, B.: Groundwater discharge creates hotspots of riparian plant species richness in a boreal forest stream network, Ecology, 95, 715–725, 2014a.
Kuglerová, L., Ågren, A., Jansson, R., and Laudon, H.: Towards optimizing riparian buffer zones: Ecological and biogeochemical implications for forest management, Forest Ecol. Manage., 334, 74–84, 2014b.
Laudon, H. and Sponseller, R. A.: How landscape organization and scale shape catchment hydrology and biogeochemistry: insights from a long-term catchment study, Wiley Interdisciplin. Rev.: Water, 5, e1265, https://doi.org/10.1002/wat2.1265, 2018.
Laudon, H., Sjöblom, V., Buffam, I., Seibert, J., and Mörth, M.: The role of catchment scale and landscape characteristics for runoff generation of boreal streams, J. Hydrol., 344, 198–209, 2007.
Laudon, H., Berggren, M., Ågren, A., Buffam, I., Bishop, K., Grabs, T., Jansson, M., and Köhler, S.: Patterns and dynamics of dissolved organic carbon (DOC) in boreal streams: the role of processes, connectivity, and scaling, Ecosystems, 14, 880–893, 2011.
Laudon, H., Taberman, I., Ågren, A., Futter, M., Ottosson-Löfvenius, M., and Bishop, K.: The Krycklan Catchment Study – a flagship infrastructure for hydrology, biogeochemistry, and climate research in the boreal landscape, Water Resour. Res., 49, 7154–7158, 2013.
Laudon, H., Seibert, J., Köhler, S., and Bishop, K.: Hydrological flow paths during snowmelt: Congruence between hydrometric measurements and oxygen 18 in meltwater, soil water, and runoff, Water Resour. Res., 40, W03102, https://doi.org/10.1029/2003WR002455, 2004a.
Laudon, H., Köhler, S., and Buffam, I.: Seasonal TOC export from seven boreal catchments in northern Sweden, Aquat. Sci., 66, 223–230, 2004b.
Lavoie, M., Paré, D., Fenton, N., Groot, A., and Taylor, K.: Paludification and management of forested peatlands in Canada: a literature review, Environ. Rev., 13, 21–50, 2005.
Leach, J., Lidberg, W., Kuglerová, L., Peralta-Tapia, A., Ågren, A., and Laudon, H.: Evaluating topography-based predictions of shallow lateral groundwater discharge zones for a boreal lake-stream system, Water Resour. Res., 53, 5420–5437, 2017.
Ledesma, J. L. J., Grabs, T., Futter, M. N., Bishop, K. H., Laudon, H., and Köhler, S. J.: Riparian zone control on base cation concentration in boreal streams, Biogeosciences, 10, 3849–3868, https://doi.org/10.5194/bg-10-3849-2013, 2013.
Ledesma, J. L. J., Grabs, T., Bishop, K. H., Schiff, S. L., and Köhler, S. J.: Potential for long-term transfer of dissolved organic carbon from riparian zones to streams in boreal catchments, Global Change Biol., 21, 2963–2979, 2015.
Ledesma, J. L. J., Kothawala, D. N., Bastviken, P., Maehder, S., Grabs, T., and Futter, M.: Stream dissolved organic matter composition reflects the riparian zone, not upslope soils in boreal forest headwaters, Water Resour. Res., 54, 3896–3912, 2018a.
Ledesma, J. L. J., Futter, M. N., Blackburn, M., Lidman, F., Grabs, T., Sponseller, R. A., Laudon, H., Bishop, K. H., and Köhler, S. J.: Towards an improved conceptualization of riparian zones in boreal forest headwaters, Ecosystems, 21, 297–315, 2018b.
Lenth, R. V.: R package lsmeans version 2.25-5, available at: http://cran.r-project.org/web/packages/lsmeans/ (last access: April 2020), 2014.
Lidman, F., Boily, Å., Laudon, H., and Köhler, S. J.: From soil water to surface water – how the riparian zone controls element transport from a boreal forest to a stream, Biogeosciences, 14, 3001–3014, https://doi.org/10.5194/bg-14-3001-2017, 2017.
Lupon, A., Denfeld, B. A., Laudon, H., Leach, J., Karlsson, J., and Sponseller, R. A.: Groundwater inflows control patterns and sources of greenhouse gas emissions from streams, Limnol. Oceanogr., 64, 1545–1557, https://doi.org/10.1002/lno.11134, 2019.
McGlynn, B. L. and McDonnell, J. J.: Quantifying the relative contributions of riparian and hillslope zones to catchment runoff, Water Resour. Res., 39, 1310, https://doi.org/10.1029/2003WR002091, 2003.
McNamara, J. P., Kane, D. L., and Hinzman, L. D.: An analysis of an arctic channel network using a digital elevation model, Geomorphology, 29, 339–353, 1999.
O'Callaghan, J. F. and Mark, D. M.: The extraction of drainage networks from digital elevation data, Comput. Vis. Graph. Image Process., 28, 323–344, 1984.
Penna, D., Van Meerveld, H., Zuecco, G., Dalla Fontana, G., and Borga, M.: Hydrological response of an Alpine catchment to rainfall and snowmelt events, J. Hydrol., 537, 382–397, 2016.
Peralta-Tapia, A., Sponseller, R. A., Ågren, A., Tetzlaff, D., Soulsby, C., and Laudon, H.: Scale-dependent groundwater contributions influence patterns of winter baseflow stream chemistry in boreal catchments, J. Geophys. Res.-Biogeo., 120, 847–858, 2015.
Ploum, S. W., Leach, J. A., Kuglerová, L., and Laudon, H.: Thermal detection of discrete riparian inflow points (DRIPs) during contrasting hydrological events, Hydrol. Process., 32, 3049–3050, 2018.
Rocher-Ros, G., Sponseller, R. A., Lidberg, W., Mörth, C.-M., and Giesler, R.: Landscape process domains drive patterns of CO2 evasion from river networks, Limnol. Oceanogr. Lett., 4, 87–95, https://doi.org/10.1002/lol2.10108, 2019.
Saarenketo, T.: Electrical properties of water in clay and silty soils, J. Appl. Geophys., 40, 73–88, 1998.
Seibert, J., Grabs, T., Köhler, S., Laudon, H., Winterdahl, M., and Bishop, K.: Linking soil- and stream-water chemistry based on a Riparian Flow-Concentration Integration Model, Hydrol. Earth Syst. Sci., 13, 2287–2297, https://doi.org/10.5194/hess-13-2287-2009, 2009.
Smith, D. G.: Effect of vegetation on lateral migration of anastomosed channels of a glacier meltwater river, Geol. Soc. Am. Bull., 87, 857–860, 1976.
Tetzlaff, D. and Soulsby, C.: Sources of baseflow in larger catchments – Using tracers to develop a holistic understanding of runoff generation, J. Hydrol., 359, 287–302, 2008.
Tiwari, T., Lundström, J., Kuglerová, L., Laudon, H., Öhman, K., and Ågren, A.: Cost of riparian buffer zones: A comparison of hydrologically adapted site-specific riparian buffers with traditional fixed widths, Water Resour. Res., 52, 1056–1069, 2016.
Tsuboyama, Y., Sidle, R. C., Noguchi, S., Murakami, S., and Shimizu, T.: A zero-order basin – Its contribution to catchment hydrology and internal hydrological processes, Hydrol. Process., 14, 387–401, 2000.
Vidon, P. G.: Not all riparian zones are wetlands: Understanding the limitation of the “wetland bias” problem, Hydrol. Process., 31, 2125–2127, 2017.
Wallace, C., McCarty, G., Lee, S., Brooks, R., Veith, T., Kleinman, P., and Sadeghi, A.: Evaluating Concentrated Flowpaths in Riparian Forest Buffer Contributing Areas Using LiDAR Imagery and Topographic Metrics, Remote Sens., 10, 614, https://doi.org/10.3390/rs10040614, 2018.