the Creative Commons Attribution 4.0 License.
the Creative Commons Attribution 4.0 License.
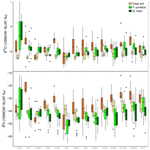
Unexplained hydrogen isotope offsets complicate the identification and quantification of tree water sources in a riparian forest
Adrià Barbeta
Sam P. Jones
Laura Clavé
Lisa Wingate
Teresa E. Gimeno
Bastien Fréjaville
Steve Wohl
We investigated plant water sources of an emblematic refugial population of Fagus sylvatica (L.) in the Ciron river gorges in south-western France using stable water isotopes. It is generally assumed that no isotopic fractionation occurs during root water uptake, so that the isotopic composition of xylem water effectively reflects that of source water. However, this assumption has been called into question by recent studies that found that, at least at some dates during the growing season, plant water did not reflect any mixture of the potential water sources. In this context, highly resolved datasets covering a range of environmental conditions could shed light on possible plant–soil fractionation processes responsible for this phenomenon. In this study, the hydrogen (δ2H) and oxygen (δ18O) isotope compositions of all potential tree water sources and xylem water were measured fortnightly over an entire growing season. Using a Bayesian isotope mixing model (MixSIAR), we then quantified the relative contribution of water sources for F. sylvatica and Quercus robur (L.) trees. Based on δ18O data alone, both species used a mix of top and deep soil water over the season, with Q. robur using deeper soil water than F. sylvatica. The contribution of stream water appeared to be marginal despite the proximity of the trees to the stream, as already reported for other riparian forests. Xylem water δ18O could always be interpreted as a mixture of deep and shallow soil waters, but the δ2H of xylem water was often more depleted than the considered water sources. We argue that an isotopic fractionation in the unsaturated zone and/or within the plant tissues could underlie this unexpected relatively depleted δ2H of xylem water, as already observed in halophytic and xerophytic species. By means of a sensitivity analysis, we found that the estimation of plant water sources using mixing models was strongly affected by this δ2H depletion. A better understanding of what causes this isotopic separation between xylem and source water is urgently needed.
- Article
(2958 KB) - Full-text XML
-
Supplement
(621 KB) - BibTeX
- EndNote
1.1 Why is an improved understanding of tree water use needed?
Ongoing climate change, through the combination of altered precipitation regimes and warmer temperatures, is affecting terrestrial ecosystems globally, promoting rapid and widespread changes in forest cover (e.g. Allen et al., 2015). This is because soil–plant interactions and species-specific water use can provoke shifts in forest species' distributions through readjustments in species abundance (Clark et al., 2016). In this context, it is a priority to better understand how spatial and temporal dynamics of water use by trees will be affected in the future. This will not only reduce uncertainties in the projections of forested areas (e.g. De Cáceres et al., 2015; Good et al., 2017) but will also improve our ecohydrological understanding of biosphere–atmosphere feedbacks and associated climate change (Berg et al., 2016; Seneviratne et al., 2013). This may also help understand how climate refugia facilitate the persistence of important biodiversity hotspots (McLaughlin et al., 2017).
1.2 The isotopic tracing method to study tree water use
Water stable isotopes (δ18O and δ2H) are commonly used as tracers of plant water use (Dawson et al., 2002; Rothfuss and Javaux, 2017; see also references below). This requires sampling all potential water sources as well as xylem water and analysing these waters for isotopic composition. Because plants can access various pools of water below ground (soil water at different depths, groundwater, rock water) but also at the soil surface (recent rain, river water), on leaf surfaces (dew) or even in the air (fog, water vapour), sampling all potential water sources can be technically challenging, destructive, expensive and/or time-consuming, and this may hamper the assessment of their temporal and spatial variability within river catchments (Fahey et al., 2017). The development of laser-based isotopic analysers in the last decade has however increased the throughput of water isotope measurements, providing opportunities to carry out observational studies at higher temporal and spatial resolution (Martín-Gómez et al., 2015).
The isotope tracing methodology is based on two main principles. Firstly (H1), it is assumed that isotopic fractionation during root water uptake (and/or xylem water redistribution) does not occur (Allison et al., 1983; Dawson and Ehleringer, 1991; Ehleringer and Dawson, 1992; White et al., 1985). Some recent studies have challenged this assumption by showing evidence of isotopic fractionation during root water uptake, but it was suggested that such an isotopic fractionation was a specific feature of saline or xeric environments (Ellsworth and Williams, 2007; Lin and Sternberg, 1993). Secondly (H2), it is essential that the isotopic compositions of all potential water sources are different enough to distinguish their relative contribution to xylem water (Ehleringer and Dawson, 1992). Processes underlying the variability in source water isotopic composition include the temporal variability in the isotopic composition of rainfall and mixing processes of water in the subsurface (Allison and Hughes, 1983; Brooks et al., 2010; Tang and Feng, 2001), the evaporative enrichment of water in surface soil layers (Allison, 1982; Sprenger et al., 2016; Tang and Feng, 2001), the seasonality of groundwater and rock moisture recharge (Oshun et al., 2015), or isotopic processes during fog water droplet formation (Scholl et al., 2011). There is no certainty however that these processes will necessarily lead to different isotopic compositions of all potential water sources. Still, if H1 is true, the δ18O and δ2H of xylem water should always lie within the range of values of all water sources.
1.3 Possible caveats of the isotopic tracing method
The water isotope tracing technique has succeeded in advancing our understanding of plant water uptake (Ehleringer and Dawson, 1992; Dawson et al., 2002). However, it occasionally leads to results that are rather unexpected. For instance, a pioneering study using hydrogen isotopes alone concluded that mature stream-side riparian trees in a semi-arid catchment did not use stream water but were dependent on an unidentified, relatively more depleted, water source, hypothesized to reflect groundwater (Dawson and Ehleringer, 1991). On the other hand, smaller trees seemed to rely on stream water. Another study conducted in a seasonally dry conifer forest in Oregon found that the δ18O and δ2H of soil and tree water were similar during the dry season and clearly distinct from stream water, even when sampled near the stream (Brooks et al., 2010). This led to the two water worlds (TWW) hypothesis whereby the first depleted rainfall water after a rainless summer fills small soil pores first and does not contribute to river flow nor to mixing with subsequent rain events, as it was already observed in ecosystems with less seasonality of rainfall (Tang and Feng, 2001). This water pool eventually participates in soil evaporation but mainly remains in the soil until being used by plant transpiration during the following dry summer. In light of this TWW hypothesis, Bowling et al. (2017) revisited the study site of Dawson and Ehleringer (1991) many years later and remeasured hydrogen but also oxygen water isotopes in xylem water, soil water at different depths and stream water, in addition to (this time) groundwater and snowmelt water. They suggested that, if the TWW were true, the soil, still dry at the end of winter, should get recharged in spring during snowmelt, leading to depleted snowpack water being locked in small soil pores and used by the trees later in the summer. Although the vertical distribution of soil water isotopes following snowmelt seemed consistent with the TWW hypothesis, neither snowmelt water nor groundwater could be identified as an alternative source for riverside trees. They concluded that the dual-isotope approach could not unambiguously determine the water sources of these riparian trees but that soil moisture seemed to be the most likely candidate, despite the proximity of the river. Oerter et al. (2019) have solved the conundrum by revisiting once more the site and sampling soil water with vapour probes. The isotopic composition of soil water vapour was similar to that of xylem water, so they could confirm that there was not a missing source. On the other hand, that may also suggest that pore-level isotopic heterogeneity interacts with root water uptake and complicates the interpretation of xylem water isotopes.
1.4 Rock moisture as an alternative plant water source?
Plant water source studies in which the xylem water isotopic composition does not spread within the range of the sources' isotopic compositions often acknowledge that a relevant source of water may not have been sampled (Bowling et al., 2017; Geris et al., 2017). Not many studies sample rock moisture, but it has been shown that water stored in rocky layers can contribute to plant transpiration, sometimes more than saturated soil layers (Barbeta and Peñuelas, 2017, and references therein). Indeed, rock moisture can represent up to 27 % of annual rainfall and can be taken up by trees during the dry season (Rempe and Dietrich, 2018). Moreover, the water stored in soil rock fragments can have an isotopic composition distinct to that of soil water or groundwater, being either relatively more depleted (in the case of δ2H in Oshun et al., 2015) or more enriched (Palacio et al., 2014; Rong et al., 2011). Such variable and contrasted isotopic effects of lithology are to be expected for differing minerals and can even cause fractionations of opposite signs for the hydrogen and oxygen isotopes (Meißner et al., 2014; Oerter et al., 2014). Thus, wherever weathered rocks constitute a large fraction of the soil volume, the isotopic composition of rock moisture should be measured as rock moisture could constitute a significant alternative plant water source.
1.5 Evidence of isotope fractionation during root water uptake
Although it cannot be ruled out that rock water in the carbonate-rich soil of Bowling et al. (2017) was a significant source of water for trees or caused any unexpected isotope effects, the very clayey soil texture reported by Brooks et al. (2010) seems less likely to contain a large rock water component. Oerter et al. (2014) showed that cations adsorbed to clay minerals create isotopically organized hydration spheres of water around them and thereby sequester these water molecules away from the bulk water. However, even if the majority of the water contained in small pores is adsorbed water that does not interact with the more mobile water (the TWW hypothesis), in summer, when only water in small pores is accessible to the trees, there should be an isotopic match between soil pore and xylem water, unless H1 is not true and isotopic fractionation occurs during root uptake. In this context, a recent controlled experiment conducted on potted avocado (Persea americana) trees has revealed isotopic fractionation during root water uptake in non-saline, relatively moist environments (Vargas et al., 2017), clearly questioning the validity of H1. Vargas et al. (2017) showed that P. americana plants discriminated against hydrogen isotopes about 10 times more than oxygen isotopes during water uptake, and this discrimination increased with soil water loss, porosity and particle size. Interestingly, the datasets reported by Brooks et al. (2010) and Oerter and Bowen (2019) contain a substantial number of xylem water samples that occupy the δ18O–δ2H space well below the soil water line, suggestive of deuterium fractionation processes during root water uptake. In fact, a growing number of studies are reporting xylem water with an isotopic composition that is relatively depleted compared to that of the considered sources (De Deurwaerder et al., 2018; Evaristo et al., 2017; Geris et al., 2017; Oerter and Bowen, 2019; Wang et al., 2017), suggesting that isotopic fractionation during root water uptake may be more common than previously thought. If such fractionation processes are not taken into account, the estimation of plant water sources may be miscalculated. The effect of an eventual deuterium fractionation on the quantification of plant water sources was addressed in a recent study by Evaristo et al. (2017). They showed that erroneous results could be obtained when a simple mass balance approach using hydrogen isotopes only was implemented, but they also concluded that results were less sensitive to deuterium fractionation when both deuterium and oxygen isotopes were combined within a Bayesian inference approach (Evaristo et al., 2017).
1.6 Aim of the study
The aim of this study was to identify the water sources of a refugial population of Fagus sylvatica (L.) in SW France, nearing the southernmost distribution limit of this species. Evidence from studies of population genetics (De Lafontaine et al., 2013) and in situ soil macrofossil charcoals radiocarbon-dated back to more than 40 kyr before present, when the area was a periglacial desert (de Lafontaine et al., 2014), indicate that the Ciron valley acted as a climate refugium during the Last Glacial Maximum (de Lafontaine et al., 2014; Timbal and Ducousso, 2010). Thereafter F. sylvatica expanded northwards and colonized the areas of its current distribution range from this and other populations in southern Europe (Gavin et al., 2014). The population is hypothesized to have persisted there since the Last Glacial Maximum (de Lafontaine et al., 2014) because of an array of edaphic, thermal and hydric features decoupled from the surrounding regional environment, notably convergent topography, frequent fog, short distances to a stream and complex lithology. In an attempt to better understand the ecohydrological mechanisms shaping this refugium, we sampled potential source waters as well as xylem water, analysed their isotopic composition and applied isotope mixing models to quantify the relative contribution of different water sources to both F. sylvatica and the more regionally widespread Quercus robur (L.). To do so, we also addressed a number of the caveats raised above including the sampling of all potential water sources, including fog and rock water, in addition to measuring both water isotopes to identify better possible isotopic fractionation during root water uptake. We used a Bayesian inference approach to quantify how plant source water varied seasonally, between species and with distance to the river. In parallel with the ecological focus of our study, the reported isotopic dataset spanning a whole growing season was also used to explore the potential effect of isotopic fractionation on the quantification of tree water sources.
2.1 Study site and experimental design
European beech (Fagus sylvatica L.) is a deciduous broadleaved tree species distributed across most of Western and Central Europe. The population that is the focus of this study is found along a mixed riparian forest on the karstic canyon formed by the Ciron, a tributary of the Garonne river, in Gironde, a south-western French region (44∘23′ N, 0∘18′ W; 60 m a.s.l.). The soil there has a fine texture and is slightly less organic than the sandy soils found in the surroundings, typical of the Aquitaine Basin (Table S1 in the Supplement). Importantly, the presence of limestone rocks weathered to various degrees creates a distinguishable carbonate-rich C horizon between 50 and 120 cm below ground (Table S1). Interestingly this European beech population is restricted either to the sheltered Ciron ravine or to slightly more distant sites (100 m) located on irregular microtopography with small karstic depressions. In this riparian forest F. sylvatica trees coexist with other deciduous species such as Quercus robur L., a regionally common tree species that dominates the canopy further away from the river. Other tree species within the riparian forest are Carpinus betulus L., Alnus glutinosa L., Corylus avellana L. and Tilia platyphyllos Scop. At the riparian forest limits beyond the chalky soil areas, we find plantations of Pinus pinaster Ait., clear cuts or agricultural fields.
The studied area has a temperate oceanic climate (Cfb in the Köppen–Geiger classification). Daily meteorological data were available from a weather station located at about 20 km from the studied site, and long-term (1897–present) monthly temperature and precipitation data were also available from another weather station located 16 km away from the studied area. Streamflow data were obtained from a stream gauge located about 4 km downstream of our study area. Over the period 1897–2015, the mean annual temperature was 12.9 ∘C and the mean annual precipitation was 813 mm yr−1, distributed rather evenly over the season. Since 1897, the mean annual temperature has increased by +1.0 ∘C (P<0.001), whereas precipitation has not showed any trend.
Early in 2017, three field plots with different conditions were set up within the riparian forest. Two of the plots were located on opposite sides of the river (NE and SW) to explore exposition effects, and a third plot, adjacent to an open area formerly occupied by a P. pinaster plantation, was chosen to explore the effect of forest fragmentation on the microclimate and notably the fog occurrence. In each of the plots, we selected five mature F. sylvatica and three Q. robur individuals of 80–150 years with all occupying dominant positions in the canopy. In addition, we selected six non-dominant F. sylvatica trees in two of the plots to explore the effect of tree size (Dawson and Ehleringer, 1991; Ehleringer and Dawson, 1992). The maximum distance between trees from the same plot was 15 m. All selected trees were sampled fortnightly from mid-April to early November 2017. In order to measure the xylem water isotopic composition, several twigs were collected from every tree, rapidly peeled to remove bark and phloem, and then placed in an airtight Exetainer® sealed with Parafilm® and kept in a cool box until they were stored in the lab at 4 ∘C. For four trees (three F. sylvatica and one Q. robur), the canopy could not be accessed and so we extracted xylem samples from coarse roots with an increment borer. Three soil cores per plot, located randomly amongst the sampled trees, were extracted with a soil auger. Each soil core was split into top soil (0–10 cm) and deep soil (from 70–80 to 110–120 cm depending on the depth of the rocky layer). While we were aware of the possibility of non-monotonic soil isotopic profiles, we considered the sampled layers as the best representatives of evaporation-exposed soil layers and deeper ones only affected by infiltrating water and subsequent mixing. This decision was done based on the characteristics of the soil profile (Table S1), in which the sampled deep soil layer had a texture that could hold most of the soil moisture, compared to upper soil layers, by having a very coarse texture and thus lower water retention capacity. Soil samples were placed in 20 mL vials with positive insert screw-top caps, sealed with Parafilm® and kept in a cool box until they were stored in the lab at 4 ∘C. From July onwards, we also sampled limestone rocks. We dug horizontally into rocky edges to avoid the effect of evaporation and collected one sample per plot and sampling date.
In addition to soil, xylem and rocks, we collected for every sampling date water from the stream, groundwater from a well located ca. 50 m from the river, and fog and rainwater from collectors installed in a small open area about 100 m away from one of our plots. Both rain and fog collectors were connected via a funnel to a thermally insulated water reservoir buried in the ground with minimal contact with the open air following the recommendations of the Global Network of Isotopes in Precipitation (GNIP) network (http://www-naweb.iaea.org/napc/ih/IHS_resources_gnip.html, last access: 1 July 2018). Each rain and fog water sample corresponds to the averaged (amount-weighted) value of the water that precipitated since the previous sampling date. The local meteoric water line (LMWL) was constructed with rainwater isotope data collected monthly since February 2007 at a GNIP station located in Cestas, France (Fig. 2). The fog collector was custom-built following the design of the single-stage Caltech Active Strand Cloud water Collector (CASCC2, Demoz et al., 1996). This design has been shown to be well suited for water isotope studies (Spiegel et al., 2012). According to the theory presented in Demoz et al. (1996) our one-stage fog collector is ill-designed to collect small fog events (i.e. clouds with droplet sizes of 7 µm or less, corresponding to a liquid water content of less than 0.01 g m−3), but such fog events are unlikely to have any significant contribution to the water source of the trees (i.e. less than 0.5 L h−1 per tree, assuming a surface of exchange of 60 m2 per tree and an average wind speed through the tree crown of 0.2 m s−1 during such fog events). On the other hand, any fog event with enough water made up of droplets larger than 7 µm in diameter was (in theory) collected, and the isotopic composition of this fraction of the cloud was expected to be representative of the entire cloud, because the isotopic composition of cloud droplets is independent of their size (Spiegel et al., 2012).
2.2 Water extraction and determination of stable isotope composition
The water contained in soil, xylem and rock samples was extracted using a cryogenic vacuum distillation system based on the design and methodology described by Orlowski et al. (2013). A detailed description of the system used is available in Jones et al. (2017). Briefly, the pressure in the extraction line was set at less than 1 Pa at the start of the extraction (i.e. when the samples were still frozen in liquid nitrogen). The samples were then gradually (within 1 h) heated up to 80 ∘C (soils) or 60 ∘C (xylem) for 2.5 h. The pressure line was continuously recorded using subatmospheric pressure sensors (APG100 Active Pirani vacuum gauges, Edwards, Burgess Hill, UK) to check that the lines remained leak-tight throughout the entire extraction. Gravimetric water content was assessed for each sample using the sample weight before and after water extraction. We also checked that the water extraction had been completed by oven drying all samples at 105 ∘C for 24 h and reweighing them.
The isotopic composition (δ2H and δ18O) of the different waters were measured with an off-axis integrated cavity optical spectrometer (TIWA-45EP, Los Gatos Research, USA) coupled to an autosampler. Details on the processing and post-correction of water samples can be found in Jones et al. (2017). The presence of organic compounds (ethanol, methanol and/or other biogenic volatile compounds) in water samples can lead to large isotopic discrepancies in laser-based analyses (Martín-Gómez et al., 2015). Organic compounds are found in certain soil types (Orlowski et al., 2018) but are more typically found in water extracted from plant tissues (Zhao et al., 2011). Post-corrections to account for the presence of organic compounds in water can be applied, based on metrics from the measured absorption spectrum (Brian Leen et al., 2012; Schultz et al., 2011). Nonetheless, these post-processing functions must be developed for each individual instrument. Following Schultz et al. (2011), we thus developed our own post-corrections by analysing Milli-Q waters mixed with methanol and/or ethanol at various concentrations and by fitting the measured deviation from the expected isotope ratio to the narrow- and broadband metrics provided by the instrument. We verified the performance of our correction with the contaminated standard WICO5 (Wassenaar et al., 2018). Xylem water samples generally exhibited higher narrow- and broadband metrics compared to rain or even soil water samples, but the corrections on xylem samples were always quite small (ca. 1.5 ‰ for δ2H and 0.7 ‰ for δ18O) compared to the correction we had to apply on the WICO5 sample, or on some of our water–alcohol mixtures used to derive our in-house post-processing functions. All isotopic data reported here are expressed on the VSMOW–SLAP scale.
2.3 Data analysis
The relationships between xylem water and its potential sources were compared at the plot level. All the analyses described below are also calculated at the plot level. Because no significant difference was found between the isotopic compositions of xylem (or water sources) between the different studied plots, “plot” was set as a random factor. To assess whether there was an isotopic offset between tree xylem water and its potential sources, the concept of the line-conditioned excess (LC-excess) proposed by Landwehr and Coplen (2006) was used: LC-excess , where a and b correspond to the slope and intercept of the LMWL, respectively. However, because the source water for a tree is more likely to be made of soil water than rainwater directly, we modified the equation above and computed the deviation of a given xylem water with respect to the soil water line (SW-excess) from the same plot and date:
where as and bs are the slope and intercept of the soil water line for a given plot and date, respectively, and δ2H and δ18O correspond to the isotopic composition of a xylem water sample collected on that plot at that date. The slope and intercept as and bs were computed by performing a linear regression on all the soil water isotope data from the surface and deep horizons collected at a given plot and date. The SW-excess of xylem water is an indicator of the δ2H offsets between xylem samples and their corresponding soil water lines. Positive SW-excess values indicate xylem samples that are more enriched in deuterium than the soil water line (and are thus positioned above soil water in a δ18O–δ2H diagram), while negative SW-excess values indicate xylem samples that are more depleted in deuterium than the soil water line (and are thus positioned below soil water in a δ18O–δ2H diagram).
The contribution of different water sources to that of xylem water was estimated using the MixSIAR package (Stock and Semmens, 2016) in R (R Core Development Team, 2012). Different mixing models were run in the script version of the package, and the number of Markov chain Monte Carlo iterations was increased manually (by trial and error) until convergence was reached and the results for the Gelman and Geweke diagnostics were acceptable. We grouped together trees of the same plot, species and date altogether and thus specified the residual error term in the isotope mixing models (Parnell et al., 2010). The potential tree water sources that we considered were restricted to the top and deep soil water and stream water/groundwater. Stream water and groundwater were pooled together as they were isotopically indistinguishable. Fog and rock moisture were not included as potential water sources with this approach because their isotopic signatures were very distant to xylem waters in a δ18O–δ2H diagram and because there were only a limited number of campaigns when they were measured. In order to test the sensitivity of MixSIAR to different data inputs, the models were run with four different types of input data: (1) δ2H and δ18O, (2) δ2H and δ18O after subtracting the SW-excess from the δ2H of xylem samples, (3) only δ18O and (4) only δ2H. Correcting xylem δ2H with SW-excess implies that tree water uptake relies only on soil water pools because the SW-excess is calculated using the slope and intercept of the soil water line. However, the lower part of this line usually overlaps with unenriched stream water and groundwater. Thus, we expected that δ2H departures from this line are meaningful in potential cases where trees are accessing not only soil water but also stream water.
The spatial, temporal, species-specific and size-related statistical comparisons between the isotopic compositions of grouped samples were analysed using linear models or, when plot and date were necessarily set as random factors, linear mixed models from the package lme4 (Bates et al., 2015) in R. For instance, for comparisons between groups across several dates, the date of sampling was set as a random factor. In order to understand the factors driving the observed SW-excess of xylem water, we fitted generalized linear mixed models (GLMM) including soil moisture (top and deep), type of sampling (coarse root or branch) soil water isotopes, tree diameter (DBH), and rainfall and vapour pressure deficit (VPD) prior to sampling and using the tree species as an explanatory variable. We selected the best model by means of the second-order Akaike information criteria (AIC). Given the water source contributions estimated with MixSIAR using different input data, we assessed their correlations with top and deep soil moisture, rainfall and VPD, also using GLMM from lme4.
3.1 Environmental conditions
The mean temperature of the 2017 (April–November) growing season was 0.4 ∘C warmer than the long-term average but 0.5 ∘C cooler than the average of the last 25 years. Precipitation during the 2017 growing season was 20 % lower than the long-term average but close to the average of the last 25 years. There was a clear deficit in precipitation from the previous winter (estimated from December 2016 to March 2017) that caused a 43 % reduction in streamflow compared to the 2000–2017 average, throughout the entire growing season (Fig. 1). Deep soil layers progressively dried over the entire growing season up to the last sampling campaign in November, while top soil moisture was usually higher but also more variable, with relatively high levels at the beginning and end of the season and levels as low as the deep soil layer only in midsummer (Fig. 1). Based on the water retention properties of top and deep soil layers, we estimated that the permanent wilting point was reached in the top soil only in early September and from late July to the end of the season in the deep soil. Using a rock density of 2.5 g cm−3, we estimated the mean volumetric water content of limestone rocks to be around 12 %, which is comparable to that of the deep soil.
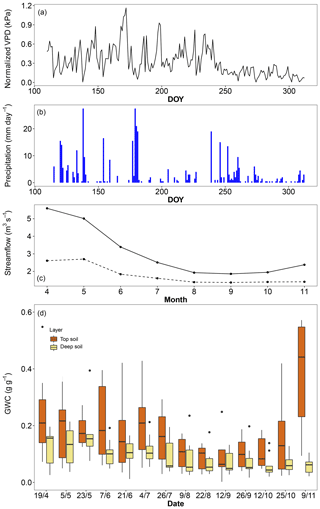
Figure 1Environmental conditions in the riparian forest along the Ciron river during the 2017 growing season. (a) Daily vapour pressure deficit (VPD), (b) daily precipitation, (c) monthly streamflow for 2017 (dashed line) and the 2010–2017 period (solid line), and (c) gravimetric water content of top soil (0–10 cm) and deep soil (ranging from 50 to 120 cm).
3.2 Stable isotopic composition of tree water sources
The long-term (2007–present) local meteoric water line using the closest GNIP station (see Material and Methods) is shown in each panel of Fig. 2. The rain data collected fortnightly plotted closely to this line and ranged, from −7.0 ‰ to −1.3 ‰ in δ18O and −46.8 ‰ to −5.4 ‰ in δ2H (Fig. 2). Fog water ranged from between −6.5 ‰ and −0.9 ‰ in δ18O and between −32.4 ‰ and −8.4 ‰ in δ2H (Fig. 2) and was not significantly different from rainwater (P>0.05 for both isotopes, Fig. S1 in the Supplement). Stream water and groundwater had isotopic compositions that were not statistically different and very stable over time ( ‰ in δ18O and ‰ in δ2H).
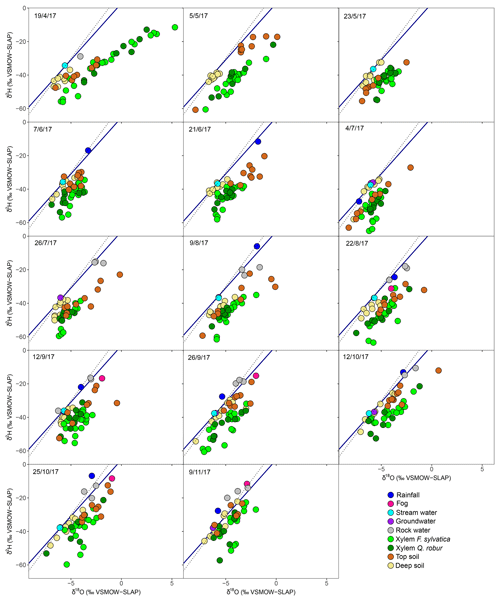
Figure 2Dual-isotope (δ2H and δ18O) plot of xylem water of the two studied species (F. sylvatica and Q. robur) and its potential sources (soil water at two depths, groundwater, stream, rain, fog and rock water) for every sampling campaign conducted in 2017. The blue line indicates the LMWL, whereas the dashed black line indicates the global meteoric water line (GMWL).
Soil water samples occupied the δ2H–δ18O space on the right side of the LMWL (Fig. 2). On average over the growing season, top soil water was significantly more enriched than deep soil (P<0.001 for both isotopes) as a result of evaporative enrichment at the soil surface. The resultant soil water line (SWL) had a mean slope of 5.17 (ranging from 4.01 to 9.99 depending on the sampling date), which is significantly smaller than the slope of the LMWL (6.73). The difference in δ18O between top and deep soil water was significantly smaller in the plot within a mixed broadleaved forest (P<0.05), suggesting that soil evaporation was probably lower at this plot.
Over the season, rainfall amounts over the 15 d preceding each sampling campaign had a negative effect on top soil water δ18O and δ2H (P<0.001) and no significant effect on the isotopic composition of the deep soil water, typical of shallow infiltration–evaporation cycles (Barnes and Allison, 1988). In the top soil, water content was negatively correlated with soil water δ18O (P<0.05) but not with δ2H. This is surprising because isotopic fractionation occurring during soil water evaporation and water vapour and liquid diffusion should affect both water isotope signals in the same direction. The fact that these water signals respond differently to top soil water content but similarly to rainfall amount (see above) indicates that observed changes in top soil water isotope signals are primarily governed by the isotopic composition of the precipitation input and only secondarily by soil water evaporative enrichment. It may also be that hydrogen isotopes of soil water are reflecting extra fractionation processes (e.g. root uptake) compared to their oxygen isotope counterparts. No similar correlation was observed between soil water content and δ18O (or δ2H) in the deep soil probably because the range of variations was smaller (Figs. 1 and 2). Finally, δ2H and δ18O of rock moisture were significantly more enriched than those of top and deep soil water but fell along the LMWL (Fig. 2). The isotopic signal of rock moisture did not differ between plots over time, nor did it correlate with weather conditions or with the isotopic signal of top or deep soil water, and thus rock soil water isotopic composition was excluded from further analyses.
3.3 Stable isotopic composition of xylem water
The isotopic composition of xylem water always fell underneath the LMWL in the dual-isotope space (Fig. 2). Xylem water from the first campaign on 19 April (i.e. just before or during budburst) was exceptionally enriched (Fig. 3) and fell in the upper right part of the dual-isotope space (top left panel in Fig. 2), except for those trees that had already flushed their leaves. This could be indicative of stem evaporative enrichment over winter, as observed in other species (Bowling et al., 2017; Martín-Gómez et al., 2017). Excluding this first campaign, xylem water samples of both F. sylvatica and Q. robur overall had a more depleted δ2H than top and deep soil water (P<0.001), as illustrated by Fig. 3. Consequently, a large number of the xylem samples fell outside the range of the considered sources in the dual-isotope space (Fig. 2).
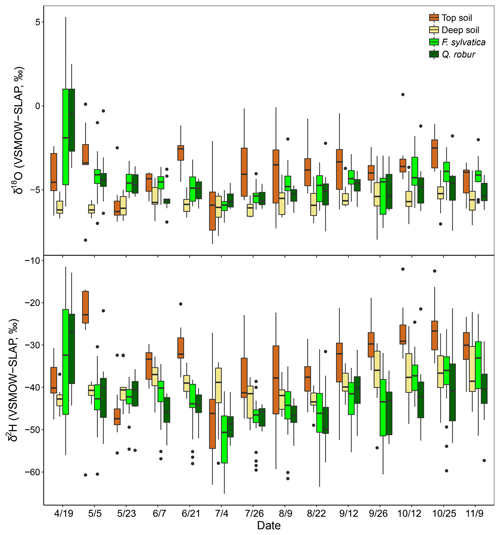
Figure 3Isotopic composition of top soil water (0–10 cm), deep soil water (50–120) and xylem water of the two studied species (F. sylvatica and Q. robur) for each sampling campaign. Data were pooled over the three studied plots. Box size represents the interquartile range, the black line is the median, the whiskers indicate variability outside the upper and lower quartiles, and individual points are outliers.
The diameters at breast height of trees (DBH) were negatively correlated with both isotopes of xylem water samples (P<0.001). Consequently, dominant trees of F. sylvatica had more depleted xylem water than non-dominant trees (P<0.01 for both isotopes). Xylem water from F. sylvatica trees presented marginally more enriched values of δ18O (P<0.05) and δ2H (P<0.1) than Q. robur trees. To our surprise, no significant differences were found in xylem water isotopes between the three studied plots. The four trees (all on the same plot) in which xylem water was extracted from outcropping coarse roots (rather than from twigs) showed a significantly more depleted δ2H over the whole season (P<0.001), but no significant difference in δ18O, compared to all the other trees (Fig. 4). The δ2H offset between xylem and soil water samples still persisted after excluding these coarse root samples, demonstrating that xylem water δ2H exhibited different patterns than δ18O.
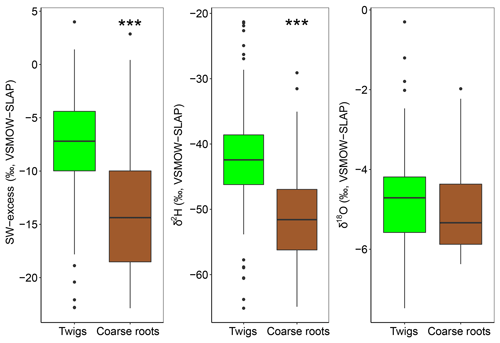
Figure 4SW-excess, δ2H and δ18O of xylem samples of dominant F. sylvatica and Q. robur depending on the part of the tree sample (twigs or coarse roots). Significant differences between twigs and coarse roots (P<0.001) are highlighted with asterisks (***). Box size represents the interquartile range, the black line is the median, the whiskers indicate variability outside the upper and lower quartiles, and individual points are outliers. Xylem samples from the first sampling campaign were excluded from the analysis because of probable winter branch evaporation (see text).
The isotopic offset between xylem and soil water samples was assessed by calculating the SW-excess. On average, xylem water samples had a SW-excess of ‰ . There were no significant differences in xylem SW-excess between species, and its seasonal variations were small (Fig. 5). Although canopy position and DBH had no effect on the SW-excess, the type of sampling had a strong influence, as the SW-excess was significantly more negative in trees whose xylem water had been sampled from coarse roots as opposed to twigs (Fig. 4, Table S2). The linear regression of the soil water line was significant for most sampling dates and plots (Table S3). Consequently, we removed from the multivariate analysis of the SW-excess those data corresponding to sampling dates and plots that did not present significant soil water line regressions. Still, the SW-excess did not significantly differ between cases with significant soil water lines and cases with non-significant soil water lines (P=0.45). Likewise, non-significant fittings of the soil water lines could entail non-monotonic isotopic soil profiles, in which intermediate layers could potentially be relatively more depleted in both isotopes than surface soil layers. In such cases, the estimation of the SW-excess could be less meaningful regarding isotopic offsets between xylem and soil water.
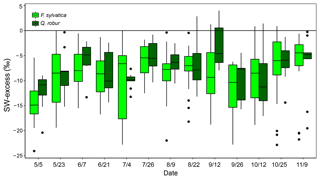
Figure 5Temporal variations of SW-excess of F. sylvatica and Q. robur xylem samples over the 2017 growing season. Box size represents the interquartile range, the black line is the median, the whiskers indicate variability outside the upper and lower quartiles, and individual points are outliers.
The GLMM used to understand the factors driving the SW-excess across time and space explained 33.3 % of the variance (Table S2). The model that best fitted the data showed that top soil water content had a positive effect on SW-excess, whereas rainfall, top soil water δ2H and daytime VPD had negative effects (larger isotopic offset between xylem water and the soil water line). The variables with larger relative importance were the type of sampling (coarse roots or twigs; see Fig. 4) and rainfall of the week prior to the sampling date.
3.4 Isotopic mixing models
The potential tree water sources that we considered were restricted to the top and deep soil water and stream water and groundwater. Stream water and groundwater were pooled together as they were isotopically indistinguishable (Fig. 2). Fog and rock moisture were not included as potential water sources because their isotopic signatures were very enriched compared to xylem water but also soil and stream water/groundwater (Fig. 2), so that their contribution would have moved xylem water samples above and to the right of the other potential sources in the dual-isotope plot, i.e. the opposite of what we observed. Also fog water could only be collected at the end of the summer, so it is unlikely to have been a significant source of water in either spring or early summer. The first set of isotopic mixing models was only run for the dominant trees of F. sylvatica and Q. robur using both δ18O and δ2H data. Because non-dominant trees were only sampled for F. sylvatica, and not for Q. robur, we preferred to exclude them when comparing the two species. On average, these mixing models indicated that F. sylvatica trees used a mix of top and deep soil water, with a marginal contribution of stream water (Fig. 6). The same mixing models also indicated that Q. robur relied mostly on soil water as well but had significantly higher contributions from stream (P<0.01) and deep soil water (P<0.01) and consequently lower contributions from top soil water (P<0.001), compared to F. sylvatica. Nonetheless, both species followed similar temporal patterns (Fig. 6). The non-dominant F. sylvatica trees also had source contributions similar to the dominant ones, although with a slight but surprisingly higher relative uptake from stream water (Fig. S2). Differences between plots were not significant (not shown).
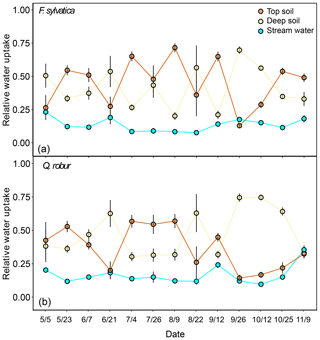
Figure 6Relative water uptake from each of the plant water sources considered: topsoil, deep soil and stream water (indistinguishable with groundwater), as estimated with MixSIAR for the dominant trees of F. sylvatica (a) and Q. robur (b). The error bars correspond to the standard deviation (N=15 for F. sylvatica and N=9 for Q. robur). These proportions were estimated with uncorrected δ2H and δ18O values for xylem water.
In a second step, we focused on the sensitivity of the isotopic mixing models to the observed δ2H offset and the dual- versus single-isotope approach. For this, we only used the isotopic data for dominant F. sylvatica trees (N=15). Correcting values of xylem δ2H for their SW-excess significantly affected the estimated source contributions of F. sylvatica (Fig. 7). The dual-isotope model with corrected δ2H values estimated a higher contribution of stream water late in the season (P<0.001) and deep soil water in the summer compared to the dual-isotope model with the original δ2H values. This was naturally accompanied by a reduction in the contribution of top soil water during summer. The single-isotope approach using only δ18O also estimated a higher contribution of stream water (P<0.001), following closely that of the deep soil, and a lower contribution of top soil water compared to the dual-isotope approach with uncorrected δ2H (Fig. 7). On the other hand, a single-isotope approach using only δ2H led to very similar contribution patterns to the dual-isotope approach with uncorrected δ2H, except at the very beginning and end of the growing season (Fig. 7).
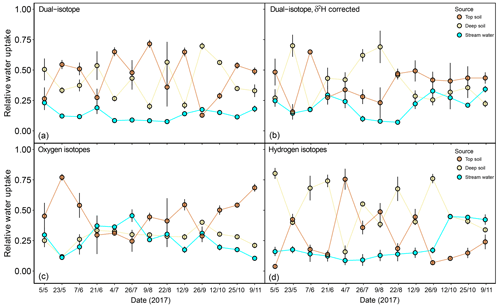
Figure 7Relative water uptake from each of the plant water sources considered: topsoil, deep soil and stream water (indistinguishable with groundwater), as estimated with MixSIAR for the dominant trees of F. sylvatica. The input data are different for each of the four panels: (a) uncorrected δ2H and δ18O, (b) δ2H (corrected for SW-excess) and δ18O, (c) only δ18O and (d) only δ2H. The error bars represent standard deviations (N=3).
The discrepancy in the estimation of source contribution to xylem water of isotope mixing models with different input data also translated into a contrasting relationship with environmental data (rainfall, VPD and soil moisture). These relationships are reported in Table 1, separated by source and input data. Overall, the models using a dual-isotope approach but with corrected δ2H values or only δ18O showed the strongest and most plausible correlations with environmental variables over the growing season. Although the contribution of stream water to xylem water estimated from δ2H only led to the best correlations with rainfall amounts and VPD, the sign of these correlations was the opposite of what is expected. That said, the use of only one isotope was not sufficient to disentangle the contribution of various water sources for some campaigns where the isotopic compositions of the different water sources were too similar (Fig. 8). In these cases, the Bayesian mixing models predicted equal contributions for each of the three water sources considered (e.g. on 4 July for δ18O only, Fig. 7).
Table 1Output of the generalized linear mixed models computed with the source contributions estimated with different input data and environmental independent variables. Dual implies the use of both water isotopes. Individual models are run per each plant water source and input data type. For each model effect, the β estimate (standardized correlation coefficient) is shown. Marginal R2 corresponds to the variance in source contribution explained by the model independent variables. Significant effects are highlighted in bold and with asterisks (* P<0.05, ** P<0.01, *** P<0.001).
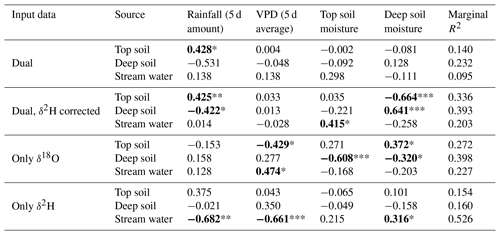
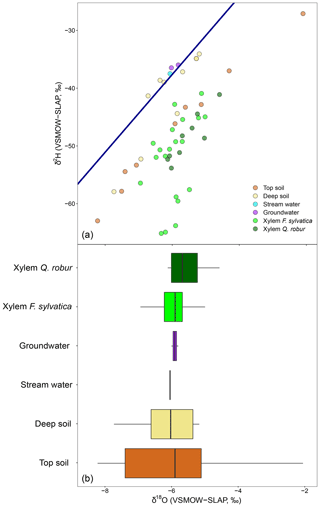
Figure 8Differences in the isotopic composition of xylem water and its potential sources using one isotope. The top panel depicts the dual-isotope plot for a single date (4 July), with xylem water and sources. The bottom panel is the box plot of the δ18O values for xylem water of F. sylvatica and Q. robur and each of the potential sources. Box size represents the interquartile range, the black line is the median, the whiskers indicate variability outside the upper and lower quartiles, and individual points are outliers.
4.1 Potential causes for the δ2H offset between xylem water and source water
Our results support those from recent studies reporting xylem water with a hydrogen isotope ratio more depleted than any of the considered water sources and thus of any of their combinations (Evaristo et al., 2017; Geris et al., 2017; Oerter and Bowen, 2019). The diversity of methodologies used for the extraction of waters and their isotopic determination in all these studies, including ours, minimises the likelihood of a common analytical or methodological bias. Furthermore, isotopic offsets measured between xylem and source water were consistent over time and space (Figs. 2 and 5). Other field datasets have shown similar isotopic offsets in semi-arid (Dawson and Ehleringer, 1991; Oerter and Bowen, 2019; Zhao et al., 2016) and saline (Lin and Sternberg, 1993) environments, but here we show that it also occurs and persists in temperate deciduous trees growing in a mild oceanic climate. Furthermore, isotopic offsets between xylem water and soil water in potted plants (Ellsworth and Williams, 2007; Vargas et al., 2017) and plants in botanical gardens (Evaristo et al., 2017) have also been reported and discussed to some extent. In addition, studies from tropical (De Deurwaerder et al., 2018), semi-arid (Wang et al., 2017), temperate (Bertrand et al., 2014; Brooks et al., 2010) and northern ecosystems (Geris et al., 2015, 2017) have also reported offsets similar in magnitude to those observed in our study. However, these results and their repercussions for partitioning were not fully discussed or explored. Our results show that δ2H offsets between xylem water and source water complicate the identification of plant water sources and the source contributions estimated by Bayesian isotopic mixing models (Fig. 7), a finding in contrast with recent studies (Evaristo et al., 2017).
The mismatch between xylem water and source water isotopes may be caused by three non-exclusive processes: (1) a water isotope separation between bound and mobile soil water (Tang and Feng, 2001; Brooks et al., 2010), (2) a water isotope fractionation occurring at the soil–root interface (Ellsworth and Stenberg, 2007; Vargas et al., 2017), or (3) a water isotope compartmentalization between vessel water and other stem water pools (Zhao et al., 2016). In particular, surface–water interactions operating at the pore level (Oerter and Bowen, 2017) and varying as a function of particle size (Gaj et al., 2017) or cation content (Oerter et al., 2014) may create isotopic heterogeneity within the soil matrix. The soil in our study site is sandy; thus the effect of interactions with clay-absorbed cations are likely to be small (Fig. S2). However, surface–water interactions on quartz silica or carbon-rich materials have also been shown to affect the water isotope composition of adsorbed water (Lin and Horita, 2016; Lin et al., 2018; Chen et al., 2016). Assuming that cryogenically extracted soil water represents bulk soil water, a disparity between adsorbed soil water and more mobile water accessible to the plant would create a mismatch between plant and bulk soil water. Indeed, probe-sampled soil water vapour appeared to be more representative of the plant-accessed soil water pool, compared to cryogenically extracted soil water (Oerter et al., 2019). But this technique seems to solve the isotopic mismatches only in some sites (Bowling et al., 2017; Oerter et al., 2019), while in others it shows a relative depletion in δ2H of xylem water, similar to that found in this study (Oerter and Bowen, 2019). In addition, adsorbed water is generally more depleted than bulk soil water (Lin et al., 2018; Lin and Horita, 2016), so the more mobile water taken up by the plants should be more enriched than bulk soil water, i.e. the opposite of what is found in this study.
Another possibility is that fractionation processes occur during water extraction. Meißner et al. (2014) reported that treating soil samples with HCl to remove carbonates prior to water extraction led to a cryogenically extracted water δ18O in agreement with that of input water, whereas the δ18O of cryogenically extracted water from carbonate-rich soil samples was depleted by about 1 ‰ compared to input water. On the other hand, they found no effect of carbonate content on hydrogen isotopes. They suggested that the δ18O depletion of extracted water was caused by oxygen isotope exchanges between soil water and carbonates during the extraction, a process that should be temperature-dependent. Meißner et al. (2014) did not specify their extraction temperature but we expect it to be >60 ∘C, i.e. close to our extraction temperature of 80 ∘C, so that we could expect a carbonate-induced isotope effect of comparable magnitude. If the presence of carbonates in the C horizon were responsible for a δ18O depletion of extracted water from the deep soil samples of about 1 ‰, this would mean that the true soil water in this horizon should be shifted by about +1 ‰. This would slightly modify the SW-excess values but would not cancel the observed isotopic offset between soil water and xylem water. Therefore, although the results of Meißner et al. (2014) are very relevant to our study, they cannot explain the isotopic offset observed here.
The water content of rocks was quite high (ca. 12 % in volume) and highly enriched in both 18O and 2H compared to bulk soil water. It is not clear what causes this enrichment of rock water compared to the surrounding soil. If root water uptake were causing it, this would mean that isotopic fractionation processes during rock water absorption by the roots enrich rock water and deplete the water taken up by the plants. Without root water uptake, the isotope composition of rock water should then be similar to the surrounding soil, and root water uptake could thus explain a depletion of plant-accessed water compared to soil water, at least when rock water contributes significantly to the plant water use. However, the resulting isotopic offset should also vary over time and space because it would be related to the soil water and rock contents at the different depths. In contrast, we measured a rather constant offset in the dual-isotope space, driven mainly by δ2H and poorly explained by environmental variables. Similar results in the literature (although scarcely discussed) can be found at sites with contrasting plant and soil types, soil moisture regimes and lithology (Brooks et al., 2010; De Deurwaerder et al., 2018; Geris et al., 2017; Vargas et al., 2017; Zhao et al., 2016). Thus, although empirical evidence for an isotope separation between bulk and plant-accessed soil water pools is growing, our results do not support that this would be produced by isotopic fractionation during rock water absorption by the plants. Otherwise, we would expect both hydrogen and oxygen isotopes to be affected and the isotope separation between plant and bulk soil waters to be weaker when soil water content is large. Instead, we found for all our trees a significant δ2H offset between xylem and soil water sources (Fig. 5), even at times when soil water content was high (Fig. 1). Similarly, we do not think that branch evaporation is responsible for the reported isotopic offset (Martín-Gómez et al., 2017). If it were the case, we would expect the magnitude of the offset to vary over the season with evaporative demand and to affect both hydrogen and oxygen isotopes, i.e. the opposite of what we report here.
The SW-excess of xylem water collected from coarse roots was significantly more depleted than that from twigs (Fig. 4). Previous studies have shown that water in coarse or tap roots can exhibit significantly lower δ2H relative to source water pools, for example in Populus euphratica (Zhao et al., 2016) and Prosopis velutina (Ellsworth and Williams, 2007). Moreover, the δ2H offsets between soil and root water observed in the former studies were of the same order of magnitude (ca. −20 ‰ for P. euphratica and ca. −7 ‰ for P. velutina) as observed here for F. sylvatica and Q. robur (Figs. 4 and 5). Interestingly, Zhao et al. (2016) were able to access plant water pools under positive pressure (using a syringe installed on the tree trunk) and found that this pool of water had the same isotopic composition as groundwater. They concluded that the depletion of bulk xylem water compared to soil water was caused by isotopic heterogeneity in the xylem water pools. In this context, Ellsworth and Williams (2007) attributed the δ2H depletion in halophytic plants to their strong dependence on the symplastic pathway of water movement into the root. Symplastic transport of water is not only important during root water uptake, but also plays a relevant role in the storage dynamics of wood water, notably during periods of increasing tension in the xylem (Pfautsch et al., 2015). This pathway uses ray parenchyma cells and is used to maintain a two-way exchange of water between the phloem and the xylem. It is thus plausible that in roots, containing a higher fraction of parenchyma cells than stems (Morris et al., 2016), a relatively higher volume of water moving through the symplast could cause a strong depletion of bulk wood water, which is the water sampled during cryogenic extraction. Interestingly, ray and axial parenchyma can account for around 31 % of total xylem tissue volume in both F. sylvatica and Q. robur (Morris et al., 2016) whilst storage water in the stem can account for up to 16 % of daily transpiration in F. sylvatica (Köcher et al., 2013) and contribute even more in some subtropical tree species (Oliva Carrasco et al., 2015). Thus future studies are now required to explore the role of symplastic water transport and storage as a potential mechanism leading to the depletion of bulk wood water δ2H compared to the actual source water signal. This mechanism may be quantitatively relevant for interpreting the isotopic composition of bulk xylem water in terms of source water and explaining the variability in SW-excess reported here.
Yet another explanation for the observed isotopic mismatch could be the development of non-monotonic isotopic profiles in the soil. Even if evaporative enrichment caused the top soil layers to be usually more depleted in both isotopes than deep soil layers (Fig. 2), it cannot be directly assumed that this isotopic gradient is gradual. Indeed, this profile is usually disrupted after rains (see 23 May and 4 July in Fig. 2), implying that surface soil layers can have a more depleted isotopic composition than deeper layers. Because we preferentially sampled deep soil at more than 70 cm depth, it is possible that the unsampled soil layers at shallower depth could hold water of similar isotopic signal than that of xylem water. Thus, the isotopic offsets between considered sources and xylem water would be caused by a not-exhaustive-enough soil water sampling. In fact, a missing water source has been sometimes claimed to explain isotopic offsets between source and xylem water (Bowling et al., 2017). However, our sampling strategy would hardly quantitatively explain our results for several reasons. Importantly, xylem δ18O was always in the range of soil water δ18O (Fig. 3). Non-monotonic soil isotopic profiles would imply that both soil water δ18O and δ2H are more depleted in a certain layer, as they should be proportional (Barbecot et al., 2018). Thus, if root water uptake was sourced in a soil layer with relatively depleted soil water, xylem water should be depleted in both isotopes, not just in δ2H. In addition, non-monotonic soil isotopic profiles may occur under some circumstances, which is probably the reason why the fit of the soil water lines changes over time and space (Table S3). However, the estimated SW-excess was negative for both species and in all sampling campaigns, i.e. following rain events and subsequent soil water percolation and isotopic mixing, short drought periods enhancing surface evaporative enrichment, and periods with a likely different depth of root water uptake. It could also be argued that depleted winter precipitation stored in middle soil layers is behind this depleted xylem water. Again, this should be reflected in both isotopes. Also, the coarse soil texture of the upper to middle soil layers (Table S1) implies fast infiltration rates, and so the sampled deep soil should in fact better reflect the isotopic composition of winter precipitation.
4.2 Estimation of source contribution to xylem water
Based on results from the Bayesian isotope mixing model with uncorrected δ18O and δ2H signals, we found that both F. sylvatica and Q. robur used a mixture of both top and deep soil water throughout the 2017 growing season, with marginal contributions of stream water (or groundwater) (Fig. 6). The relative contribution of these water sources was also quite dynamic, leading for example to rapid shifts towards the deep soil in late summer when soil was progressively drying. According to this analysis, we also found that Q. robur used more deep soil water than F. sylvatica, which is consistent with the idea that oaks tend to invest more in deep roots (Rosengren et al., 2005), whilst beech trees usually have a dense fine root network in top soil layers (Leuschner et al., 2001). When using δ18O alone or in combination with SW-excess-corrected δ2H signals, the contribution of stream water/groundwater to the water sources of F. sylvatica trees increased significantly (Fig. 7). Typically, soils in this region are acidic and sandy and contain a hardpan that restricts access to the groundwater. In contrast, the riparian forest in the present study was located on a slightly more basic soil (Table S1) with a rocky layer of limestone that provided a water connection to the groundwater. This may be a reason why F. sylvatica is consistently restricted to a few particular sites in its southern range (De Lafontaine et al., 2014; Timbal and Ducousso, 2010).
Studies applying isotope mixing models such as MixSIAR to study plant water sources usually assume no isotopic offset between xylem water and source water (Barbeta et al., 2015; Evaristo et al., 2017; Palacio et al., 2014; Rothfuss and Javaux, 2017). However, we have shown evidence that δ2H offsets are present as a result of isotopic fractionation processes either within soil water pools or within plant tissues, and we made an attempt to evaluate their effects on the estimates of plant water sources by comparing mixing models with different input data. This exercise was made with the purpose of providing a sensitivity analysis. However, its use in other sites and plant species should be carried out with caution, as it is very likely that the observed δ2H offset may display different patterns depending on other water sources. By correcting xylem δ2H based on the SW-excess, we found that the contribution of stream water had been underestimated compared with the classical approach (Fig. 7). Contrary to recent studies that reported a low sensitivity of Bayesian isotope mixing models to δ2H offsets (Evaristo et al., 2017), we found that the plant water source estimations also varied between models using either δ18O only, δ2H only or models using both isotopes. In addition, this disparity caused by δ2H offsets cannot be solved by using only the apparently non-fractionating δ18O as having one isotopic tracer is insufficient to distinguish between water sources in many cases (Fig. 8).
Bayesian mixing models were shown to perform best in a recent comparison of approaches to quantify root water uptake (Rothfuss and Javaux, 2017). These comparisons were based however on the assumption that no fractionation occurs during root water uptake and redistribution within the plant tissues. Therefore, the application of these models may not be suitable in studies where δ2H offsets are suspected. Interestingly, we found that correcting the xylem δ2H data using the SW-excess gave stronger correlations with environmental data and allowed for a more parsimonious interpretation, such as an increase in top soil water uptake with cumulative 5 d rainfall amounts (Table 1). Based on these correlations, such a correction using the SW-excess appeared to improve the predictive power of the dual-isotope approach. However, correcting systematically the xylem water isotopic data with the SW-excess should not be done in cases where water sources apart from soil water are suspected to contribute to xylem water. Finally, spatiotemporal dynamics in the soil water isotope profile could also complicate the concept of the soil water isotope line and thus the SW-excess. The fact that we sometimes observed positive SW-excess indicates that we do not only correct for one single fractionation factor and demonstrates the limitation of the SW-excess correction proposed here. In addition, in our study, the correlation of soil water δ18O and δ2H was not always significant (Table S3), although this did not seem to affect the SW-excess quantitatively. However, the concept of the SW-excess becomes less meaningful when soil water isotopes are not significantly correlated, since the error associated with the regression coefficients could be of the same magnitude as that of the calculated SW-excess.
In the light of our results and other recent studies, either conducted under controlled conditions (Oerter et al., 2014; Vargas et al., 2017) or in the field (Evaristo et al., 2017; Oerter and Bowen, 2017; Oshun et al., 2015; Zhao et al., 2016), evidence for fractionation processes occurring at the soil–root interface or within plant woody tissues is growing. These processes may complicate or prevent the identification of plant water sources, especially when they remain unnoticed. Importantly, δ2H fractionation during or after root water uptake seems to extend beyond plants growing in salty and dry environments (Ellsworth and Williams, 2007). This should now motivate researchers to develop hypothesis-driven studies focused on two main lines: firstly, to couple the study of physicochemical fractionation processes in the unsaturated zone and their repercussions on plant-absorbed water, covering a range of soil properties and water content (as illustrated by Vargas et al., 2017); secondly, to obtain a better understanding of the isotopic dynamics of water pools within plant tissues, notably those associated with plant storage water and its dynamics (Pfautsch et al., 2015), which will require developing new extraction methods for xylem water (e.g. a method allowing us to separate younger xylem vessel water from older water stored outside the xylem; see Zhao et al., 2016). Interestingly, if there are isotopically distinct water pools within plant stems, this could be used to quantify the contribution and age of wood water storage. On the other hand, this would clearly complicate the use of Bayesian isotope mixing models to partition plant water sources. Nonetheless a better understanding of what causes this isotopic separation between xylem and source water is urgently needed to constrain their respective contributions in isotope mixing models, as already applied in other applications such as in food web studies (Phillips et al., 2014), and provide more parsimonious plant water source estimations.
Although the present dataset does not allow us to assess definitively which are the ecohydrological mechanisms that have assisted the persistence of F. sylvatica in this riparian forest for at least the last 40 000 years, we can rule out a dominance of water uptake from the stream, as found in other riparian tree species (Bowling et al., 2017; Dawson and Ehleringer, 1991; Oerter et al., 2019). We cannot rule out that the contribution of stream or groundwater, although small, is responsible for the long-term persistence of F. sylvatica in this area, but the presence of a layer of weathered limestone seems also to confer advantageous soil characteristics to F. sylvatica. Indeed, the C horizon is located within the rooting depth of both studied species (at a depth of 50–120 cm) and has higher clay and finer sand fractions than the A and B horizons and than soils in the surrounding region. Our findings that this (deep soil) C horizon seems to contribute significantly to the water source of beech trees indicate that the higher water holding capacity of soils around the Ciron river could be responsible for the long-term persistence of F. sylvatica in this valley. It is worth noting that, although 2017 was characterized by relatively low soil water content early in the season, the precipitation input was evenly spread over the growing season and the atmospheric evaporative demand did not stay high for prolonged periods (Fig. 1). Therefore, it would be interesting to perform a similar survey during a year with a prolonged drought and high evaporative demand (e.g. in the top percentile hotter and dryer years of the last 25 years) to observe how the contribution of ground or river water and deep soil water varied in these years. This would be necessary in order to perform projections of the persistence likelihood of F. sylvatica in this valley exposed to a potentially drier climate in the future.
The data collected in this study are available upon request to the authors.
The supplement related to this article is available online at: https://doi.org/10.5194/hess-23-2129-2019-supplement.
AB, JO and LW designed the study. AB, LC, BF, SPJ, SW and TEG conducted the field work. SPJ, SW, JO and LW developed and tested the cryogenic water extraction vacuum line and produced the protocols and code to process water isotope data. BF and LC ran all the samples through the water extraction line. AB, LC, BF and SPJ conducted the water isotope analyses. AB and JO analysed the data and wrote a first draft of the manuscript. All the other authors edited the manuscript in several rounds of revision.
The authors declare that they have no conflict of interest.
This study has been carried out on the HydroBeech, ClimBeech and MicroMic projects with financial support from the French National Research Agency (ANR) in the frame of the Investments for the Future Programme, within the Cluster of Excellence COTE (ANR-10-LABX-45). Adrià Barbeta acknowledges an IdEx Bordeaux postdoctoral fellowship from the Université de Bordeaux (contract no. 22001162). This project has also received funding from the European Research Council (ERC) under the European Union's Seventh Framework Programme (FP7/2007-2013) (grant agreement no. 338264) awarded to Lisa Wingate, a Marie Skłodowska-Curie Intra-European Fellowship (grant agreement no. 653223) awarded to Teresa E. Gimeno, the French Agence National de la Recherche (ANR) (grant agreement no. ANR-13-BS06-0005-01) awarded to Jérôme Ogée and the Aquitaine Region project Athene awarded to Lisa Wingate (2016-1R20301-00007218).
This paper was edited by Lixin Wang and reviewed by Xin Song and Juan Ferrio.
Allen, C. D., Breshears, D. D., McDowell, N. G., Llen, C. R. D. A., Reshears, D. A. D. B., Allen, C. D., Breshears, D. D., McDowell, N. G., Llen, C. R. D. A., Reshears, D. A. D. B., Allen, C. D., Breshears, D. D., and McDowell, N. G.: On underestimation of global vulnerability to tree mortality and forest die-off from hotter drought in the Anthropocene, Ecosphere, 6, 1–55, https://doi.org/10.1890/ES15-00203.1, 2015.
Allison, G. B.: The relationship between 18O and deuterium in water in sand columns undergoing evaporation, J. Hydrol., 55, 163–169, 1982.
Allison, G. B. and Hughes, M. W.: The use of natural tracers as indicators of soil-water movement in a temperate semi-arid region, J. Hydrol., 60, 157–173, https://doi.org/10.1016/0022-1694(83)90019-7, 1983.
Allison, G. B., Barnes, C. J., Hugues, M. W., and Leaney, F. W. J.: Effect of Climate and Vegetation on Oxygen-18 and Deuterium Profiles in Soils, in: Isotope hydrology, 1983: proceedings of an International Symposium on Isotope Hydrology in Water Resources Development, 105–123, International Atomic Energy Agency, Vienna, available at: https://inis.iaea.org/search/search.aspx?orig_q=RN:15067096 (last access: 1 July 2018), 1983.
Barbecot, F., Schneider, V., Larocque, M., Pili, E., Gibert-Brunet, E., Hélie, J.-F., Plain, C., Meyzonnat, G., Noret, A., Guillon, S., and Mattei, A.: Using Water Stable Isotopes in the Unsaturated Zone to Quantify Recharge in Two Contrasted Infiltration Regimes, Vadose Zo. J., 17, 170170, https://doi.org/10.2136/vzj2017.09.0170, 2018.
Barbeta, A. and Peñuelas, J.: Relative contribution of groundwater to plant transpiration estimated with stable isotopes, Sci. Rep.-UK, 7, 10580, https://doi.org/10.1038/s41598-017-09643-x, 2017.
Barbeta, A., Mejía-Chang, M., Ogaya, R., Voltas, J., Dawson, T. E., and Peñuelas, J.: The combined effects of a long-term experimental drought and an extreme drought on the use of plant-water sources in a Mediterranean forest, Glob. Chang. Biol., 21, 1213–1225, https://doi.org/10.1111/gcb.12785, 2015.
Barnes, C. J. and Allison, G. B.: Tracing of water movement in the unsaturated zone using stable isotopes of hydrogen and oxygen, J. Hydrol., 100, 143–176, https://doi.org/10.1371/journal.pone.0195301, 1988.
Bates, D., Mächler, M., Bolker, B., and Walker, S.: Fitting Linear Mixed-Effects Models using lme4, J. Stat. Softw., 67, 1–48, https://doi.org/10.18637/jss.v067.i01, 2015.
Berg, A., Findell, K., Lintner, B., Giannini, A., Seneviratne, S. I., Van Den Hurk, B., Lorenz, R., Pitman, A., Hagemann, S., Meier, A., Cheruy, F., Ducharne, A., Malyshev, S., and Milly, P. C. D.: Land-atmosphere feedbacks amplify aridity increase over land under global warming, Nat. Clim. Chang., 6, 869–874, https://doi.org/10.1038/nclimate3029, 2016.
Bertrand, G., Masini, J., Goldscheider, N., Meeks, J., Lavastre, V., Celle-Jeanton, H., Gobat, J. M., and Hunkeler, D.: Determination of spatiotemporal variability of tree water uptake using stable isotopes (δ18O, δ2H) in an alluvial system supplied by a high-altitude watershed, Pfyn forest, Switzerland, Ecohydrology, 7, 319–333, https://doi.org/10.1002/eco.1347, 2014.
Bowling, D. R., Schulze, E. S., and Hall, S. J.: Revisiting streamside trees that do not use stream water: can the two water worlds hypothesis and snowpack isotopic effects explain a missing water source?, Ecohydrology, 10, 1–12, https://doi.org/10.1002/eco.1771, 2017.
Brian Leen, J., Berman, E. S. F., Liebson, L., and Gupta, M.: Spectral contaminant identifier for off-axis integrated cavity output spectroscopy measurements of liquid water isotopes, Rev. Sci. Instrum., 83, 044305, https://doi.org/10.1063/1.4704843, 2012.
Brooks, J. R., Barnard, H. R., Coulombe, R., McDonnell, J. J., Renée Brooks, J., Barnard, H. R., Coulombe, R., and McDonnell, J. J.: Ecohydrologic separation of water between trees and streams in a Mediterranean climate, Nat. Geosci., 3, 100–104, https://doi.org/10.1038/ngeo722, 2010.
Chen, G., Auerswald, K., and Schnyder, H.: 2H and 18O depletion of water close to organic surfaces, Biogeosciences, 13, 3175–3186, https://doi.org/10.5194/bg-13-3175-2016, 2016.
Clark, J. S., Iverson, L., Woodall, C. W., Allen, C. D., Bell, D. M., Bragg, D. C., D'Amato, A. W., Davis, F. W., Hersh, M. H., Ibanez, I., Jackson, S. T., Matthews, S., Pederson, N., Peters, M., Schwartz, M. W., Waring, K. M., and Zimmermann, N. E.: The impacts of increasing drought on forest dynamics, structure, and biodiversity in the United States, Glob. Chang. Biol., 22, 2329–2352, https://doi.org/10.1111/gcb.13160, 2016.
Dawson, T. E. and Ehleringer, J. R.: Streamside trees that do not use stream water, Nature, 350, 335–337, 1991.
Dawson, T. E., Mambelli, S., Plamboeck, A. H., Templer, P. H., and Tu, K. P.: Stable Isotopes in Plant Ecology, Annu. Rev. Ecol. Syst., 33, 507–559, https://doi.org/10.1146/annurev.ecolsys.33.020602.095451, 2002.
De Cáceres, M., Martínez-Vilalta, J., Coll, L., Llorens, P., Casals, P., Poyatos, R., Pausas, J. G., and Brotons, L.: Coupling a water balance model with forest inventory data to predict drought stress: The role of forest structural changes vs. climate changes, Agr. Forest Meteorol., 213, 77–90, https://doi.org/10.1016/j.agrformet.2015.06.012, 2015.
De Deurwaerder, H., Hervé-Fernández, P., Stahl, C., Burban, B., Petronelli, P., Hoffman, B., Bonal, D., Boeckx, P., and Verbeeck, H.: Liana and tree below-ground water competition – evidence for water resource partitioning during the dry season, Tree Physiol., 38, 1–13, https://doi.org/10.1093/treephys/tpy002, 2018.
De Lafontaine, G., Ducousso, A., Lefèvre, S., Magnanou, E., and Petit, R. J.: Stronger spatial genetic structure in recolonized areas than in refugia in the European beech, Mol. Ecol., 22, 4397–4412, https://doi.org/10.1111/mec.12403, 2013.
de Lafontaine, G., Amasifuen Guerra, C. A., Ducousso, A., Petit, R. J., Lafontaine, G. De, Alberto, C., Guerra, A., Ducousso, A., and Petit, J.: Cryptic no more: Soil macrofossils uncover Pleistocene forest microrefugia within a periglacial desert, New Phytol., 204, 715–729, https://doi.org/10.1111/nph.12833, 2014.
Demoz, B. B., Collett, J. L., and Daube, B. C.: On the caltech active strand cloudwater collectors, Atmos. Res., 41, 47–62, https://doi.org/10.1016/0169-8095(95)00044-5, 1996.
Ehleringer, J. R. R. and Dawson, T. E.: Water uptake by plants: perspectives from stable isotope composition, Plant Cell Environ., 15, 1073–1082, https://doi.org/10.1111/j.1365-3040.1992.tb01657.x, 1992.
Ellsworth, P. Z. and Williams, D. G.: Hydrogen isotope fractionation during water uptake by woody xerophytes, Plant Soil, 291, 93–107, https://doi.org/10.1007/s11104-006-9177-1, 2007.
Evaristo, J., McDonnell, J. J., and Clemens, J.: Plant source water apportionment using stable isotopes: A comparison of simple linear, two-compartment mixing model approaches, Hydrol. Process., 31, 3750–3758, https://doi.org/10.1002/hyp.11233, 2017.
Fahey, T. J., Yanai, R. D., Gonzales, K. E., and Lombardi, J. A.: Sampling and processing roots from rocky forest soils, Ecosphere, 8, e01863, https://doi.org/10.1002/ecs2.1863, 2017.
Gaj, M., Kaufhold, S., Koeniger, P., Beyer, M., Weiler, M., and Himmelsbach, T.: Mineral mediated isotope fractionation of soil water, Rapid Commun. Mass Spectrom., 31, 269–280, https://doi.org/10.1002/rcm.7787, 2017.
Gavin, D. G., Fitzpatrick, M. C., Gugger, P. F., Heath, K. D., Dobrowski, S. Z., Hampe, A., Hu, F. S., Ashcroft, M. B., Bartlein, P. J., Blois, J. L., Carstens, B. C., Davis, E. B., Lafontaine, G. De, Edwards, M. E., Fernandez, M., Henne, P. D., Herring, E. M., Tsai, Y. E., and Williams, J. W.: Climate refugia?: joint inference from fossil records , species distribution models and phylogeography, New Phytol., 204, 37–54, 2014.
Geris, J., Tetzlaff, D., McDonnell, J., Anderson, J., Paton, G., and Soulsby, C.: Ecohydrological separation in wet, low energy northern environments? A preliminary assessment using different soil water extraction techniques, Hydrol. Process., 29, 5139–5152, https://doi.org/10.1002/hyp.10603, 2015.
Geris, J., Tetzlaff, D., McDonnell, J. J., and Soulsby, C.: Spatial and temporal patterns of soil water storage and vegetation water use in humid northern catchments, Sci. Total Environ., 595, 486–493, https://doi.org/10.1016/j.scitotenv.2017.03.275, 2017.
Good, S. P., Moore, G. W., and Miralles, D. G.: A mesic maximum in biological water use demarcates biome sensitivity to aridity shifts, Nat. Ecol. Evol., 1, 1883–1888, https://doi.org/10.1038/s41559-017-0371-8, 2017.
Jones, S. P., Ogée, J., Sauze, J., Wohl, S., Saavedra, N., Fernández-Prado, N., Maire, J., Launois, T., Bosc, A., and Wingate, L.: Non-destructive estimates of soil carbonic anhydrase activity and associated soil water oxygen isotope composition, Hydrol. Earth Syst. Sci., 21, 6363–6377, https://doi.org/10.5194/hess-21-6363-2017, 2017.
Köcher, P., Horna, V., and Leuschner, C.: Stem water storage in five coexisting temperate broad-leaved tree species: Significance, temporal dynamics and dependence on tree functional traits, Tree Physiol., 33, 817–832, https://doi.org/10.1093/treephys/tpt055, 2013.
Landwehr, J. M. and Coplen, T. B.: Line-conditioned excess: a new method for characterizing stable hydrogen and oxygen isotope ratios in hydrologic systems, in: Isotopes in Environmental Studies Isotopes in Environmental Studies, 132–135, International Atomic Energy Agency, Vienna, 2006.
Leuschner, C., Hertel, D., Coners, H., and Büttner, V.: Root competition between beech and oak: A hypothesis, Oecologia, 126, 276–284, https://doi.org/10.1007/s004420000507, 2001.
Lin, G. and Sternberg, L. da S. L.: Hydrogen isotopic fractionation by plant roots during water uptake in coastal wetland plants, in: Stable isotopes and plant carbon-water relations, edited by: Ehleringer, J., Hall, A., and Farquhar, G., 497–510, Academic Press Inc., New York, 1993.
Lin, Y. and Horita, J.: An experimental study on isotope fractionation in a mesoporous silica-water system with implications for vadose-zone hydrology, Geochim. Cosmochim. Ac., 184, 257–271, https://doi.org/10.1016/j.gca.2016.04.029, 2016.
Lin, Y., Horita, J., and Abe, O.: Adsorption isotope effects of water on mesoporous silica and alumina with implications for the land-vegetation-atmosphere system, Geochim. Cosmochim. Ac., 223, 520–536, https://doi.org/10.1016/j.gca.2017.12.021, 2018.
Martín-Gómez, P., Barbeta, A., Voltas, J., Peñuelas, J., Dennis, K., Palacio, S., Dawson, T. E., and Ferrio, J. P.: Isotope-ratio infrared spectroscopy: A reliable tool for the investigation of plant-water sources?, New Phytol., 207, 914–927, https://doi.org/10.1111/nph.13376, 2015.
Martín-Gómez, P., Serrano, L., and Ferrio, J. P.: Short-term dynamics of evaporative enrichment of xylem water in woody stems: Implications for ecohydrology, Tree Physiol., 37, 511–522, https://doi.org/10.1093/treephys/tpw115, 2017.
McLaughlin, B. C., Ackerly, D. D., Klos, P. Z., Natali, J., Dawson, T. E., and Thompson, S. E.: Hydrologic refugia, plants, and climate change, Glob. Chang. Biol., 23, 2941–2961, https://doi.org/10.1111/gcb.13629, 2017.
Meißner, M., Köhler, M., Schwendenmann, L., Hölscher, D., and Dyckmans, J.: Soil water uptake by trees using water stable isotopes (δ2H and δ18O) – a method test regarding soil moisture, texture and carbonate, Plant Soil, 376, 327–335, https://doi.org/10.1007/s11104-013-1970-z, 2014.
Morris, H., Plavcová, L., Cvecko, P., Fichtler, E., Gillingham, M. A. F., Martínez-Cabrera, H. I., Mcglinn, D. J., Wheeler, E., Zheng, J., Ziemińska, K., and Jansen, S.: A global analysis of parenchyma tissue fractions in secondary xylem of seed plants, New Phytol., 209, 1553–1565, https://doi.org/10.1111/nph.13737, 2016.
Oerter, E. J. and Bowen, G.: In situ monitoring of H and O stable isotopes in soil water reveals ecohydrologic dynamics in managed soil systems, Ecohydrology, 10, e1841, https://doi.org/10.1002/eco.1841, 2017.
Oerter, E. J. and Bowen, G. J.: Spatiotemporal heterogeneity in soil water stable isotopic composition and its ecohydrologic implications in semi-arid ecosystems, Hydrol. Process., 0–2, https://doi.org/10.1002/hyp.13434, 2019.
Oerter, E., Finstad, K., Schaefer, J., Goldsmith, G. R., Dawson, T., and Amundson, R.: Oxygen isotope fractionation effects in soil water via interaction with cations (Mg, Ca, K, Na) adsorbed to phyllosilicate clay minerals, J. Hydrol., 515, 1–9, https://doi.org/10.1016/j.jhydrol.2014.04.029, 2014.
Oerter, E. J., Siebert, G., Bowling, D. R., and Bowen, G.: Soil water vapor isotopes identify missing water source for streamside trees, Ecohydrology, e2083, https://doi.org/10.1002/eco.2083, 2019.
Oliva Carrasco, L., Bucci, S. J., Di Francescantonio, D., Lezcano, O. A., Campanello, P. I., Scholz, F. G., Rodríguez, S., Madanes, N., Cristiano, P. M., Hao, G. Y., Holbrook, N. M., and Goldstein, G.: Water storage dynamics in the main stem of subtropical tree species differing in wood density, growth rate and life history traits, Tree Physiol., 35, 354–365, https://doi.org/10.1093/treephys/tpu087, 2015.
Orlowski, N., Frede, H.-G., Brüggemann, N., and Breuer, L.: Validation and application of a cryogenic vacuum extraction system for soil and plant water extraction for isotope analysis, J. Sens. Sens. Syst., 2, 179–193, https://doi.org/10.5194/jsss-2-179-2013, 2013.
Orlowski, N., Breuer, L., Angeli, N., Boeckx, P., Brumbt, C., Cook, C. S., Dubbert, M., Dyckmans, J., Gallagher, B., Gralher, B., Herbstritt, B., Hervé-Fernández, P., Hissler, C., Koeniger, P., Legout, A., Macdonald, C. J., Oyarzún, C., Redelstein, R., Seidler, C., Siegwolf, R., Stumpp, C., Thomsen, S., Weiler, M., Werner, C., and McDonnell, J. J.: Inter-laboratory comparison of cryogenic water extraction systems for stable isotope analysis of soil water, Hydrol. Earth Syst. Sci., 22, 3619–3637, https://doi.org/10.5194/hess-22-3619-2018, 2018.
Oshun, J., Dietrich, W. E., Dawson, T. E., and Fung, I.: Dynamic, structured heterogeneity of water isotopes inside hillslopes, Water Resour. Res., 52, 4840–4847, https://doi.org/10.1002/2015WR017485, 2015.
Palacio, S., Azorín, J., Montserrat-Martí, G., and Ferrio, J. P.: The crystallization water of gypsum rocks is a relevant water source for plants, Nat. Commun., 5, 4660, https://doi.org/10.1038/ncomms5660, 2014.
Parnell, A. C., Inger, R., Bearhop, S., and Jackson, A. L.: Source partitioning using stable isotopes: coping with too much variation, PLoS One, 5, e9672, https://doi.org/10.1371/journal.pone.0009672, 2010.
Pfautsch, S., Renard, J., Tjoelker, M. G., and Salih, A.: Phloem as Capacitor: Radial Transfer of Water into Xylem of Tree Stems Occurs via Symplastic Transport in Ray Parenchyma, Plant Physiol., 167, 963–971, https://doi.org/10.1104/pp.114.254581, 2015.
Phillips, D. L., Inger, R., Bearhop, S., Jackson, A. L., Moore, J. W., Parnell, A. C., Semmens, B. X., and Ward, E. J.: Best practices for use of stable isotope mixing models in, Can. J. Zool., 835, 823–835, https://doi.org/10.1139/cjz-2014-0127, 2014.
R Core Development Team: R: A language and environment for statistical computing. R Foundation for Statistical Computing, available at: http://www.r-project.org/ (last access: 2 April 2017), 2012.
Rempe, D. M. and Dietrich, W. E.: Direct observations of rock moisture, a hidden component of the hydrologic cycle, P. Natl. Acad. Sci. USA, 115, 2664–2669, https://doi.org/10.1073/pnas.1800141115, 2018.
Rong, L., Chen, X., Chen, X., Wang, S., and Du, X.: Isotopic analysis of water sources of mountainous plant uptake in a karst plateau of southwest China, Hydrol. Process., 25, 3666–3675, https://doi.org/10.1002/hyp.8093, 2011.
Rosengren, U., Göransson, H., Jönsson, U., Stjernquist, I., Thelin, G., and Wallander, H.: Functional Biodiversity Aspects on the Nutrient Sustainability in Forests-Importance of Root Distribution, J. Sustain. For., 21, 77–100, https://doi.org/10.1186/1472-6785-11-29, 2005.
Rothfuss, Y. and Javaux, M.: Reviews and syntheses: Isotopic approaches to quantify root water uptake: a review and comparison of methods, Biogeosciences, 14, 2199–2224, https://doi.org/10.5194/bg-14-2199-2017, 2017.
Scholl, M., Eugster, W., and Burkard, R.: Understanding the role of fog in forest hydrology: Stable isotopes as tools for determining input and partitioning of cloud water in montane forests, Hydrol. Process., 25, 353–366, https://doi.org/10.1002/hyp.7762, 2011.
Schultz, N. M., Griffis, T. J., Lee, X., and Baker, J. M.: Identification and correction of spectral contamination in 2H∕1H and 18O∕16O measured in leaf, stem, and soil water, Rapid Commun. Mass Spectrom., 25, 3360–3368, https://doi.org/10.1002/rcm.5236, 2011.
Seneviratne, S. I., Wilhelm, M., Stanelle, T., Van Den Hurk, B., Hagemann, S., Berg, A., Cheruy, F., Higgins, M. E., Meier, A., Brovkin, V., Claussen, M., Ducharne, A., Dufresne, J. L., Findell, K. L., Ghattas, J., Lawrence, D. M., Malyshev, S., Rummukainen, M., and Smith, B.: Impact of soil moisture-climate feedbacks on CMIP5 projections: First results from the GLACE-CMIP5 experiment, Geophys. Res. Lett., 40, 5212–5217, https://doi.org/10.1002/grl.50956, 2013.
Spiegel, J. K., Zieger, P., Bukowiecki, N., Hammer, E., Weingartner, E., and Eugster, W.: Evaluating the capabilities and uncertainties of droplet measurements for the fog droplet spectrometer (FM-100), Atmos. Meas. Tech., 5, 2237–2260, https://doi.org/10.5194/amt-5-2237-2012, 2012.
Sprenger, M., Leistert, H., Gimbel, K., and Weiler, M.: Illuminating hydrological processes at the soil-vegetation-atmosphere interface with water stable isotopes, Rev. Geophys., 54, 674–704, https://doi.org/10.1002/2015RG000515, 2016.
Stock, B., Jackson, A., Ward, E., and Venkiteswaran, J.: brianstock/MixSIAR 3.1.9 (Version 3.1.9), Zenodo, https://doi.org/10.5281/zenodo.1209993, 2018.
Tang, K. and Feng, X.: The effect of soil hydrology on the oxygen and hydrologen isotopic compositions of plants' source water, Earth Planet. Sc. Lett., 185, 355–367, https://doi.org/10.1016/S0012-821X(00)00385-X, 2001.
Timbal, J. and Ducousso, A.: Le Hêtre (Fagus sylvatica L.) dans les Landes de Gascogne et à leur périphérie, Bulletin de la Société Linnéenne de Bordeaux, 38, 127–137, 2010.
Vargas, A. I., Schaffer, B., Yuhong, L., and Sternberg, L. da S. L.: Testing plant use of mobile vs immobile soil water sources using stable isotope experiments, New Phytol., 215, 582–594, https://doi.org/10.1111/nph.14616, 2017.
Wang, J., Fu, B., Lu, N., and Zhang, L.: Seasonal variation in water uptake patterns of three plant species based on stable isotopes in the semi-arid Loess Plateau, Sci. Total Environ., 609, 27–37, https://doi.org/10.1016/j.scitotenv.2017.07.133, 2017.
White, J., Cook, E., Lawrence, J., and Broecker, W.: The D/H Ratios of Sap in Trees - Implications for Water Sources and Tree-Ring D/H Ratios, Geochim. Cosmochim. Ac., 49, 237–246, https://doi.org/10.1016/0016-7037(85)90207-8, 1985.
Zhao, L., Xiao, H., Zhou, J., Wang, L., Cheng, G., Zhou, M., Yin, L., and McCabe, M. F.: Detailed assessment of isotope ratio infrared spectroscopy and isotope ratio mass spectrometry for the stable isotope analysis of plant and soil waters, Rapid Commun. Mass Spectrom., 25, 3071–3082, https://doi.org/10.1002/rcm.5204, 2011.
Zhao, L., Wang, L., Cernusak, L. A., Liu, X., Xiao, H., Zhou, M., and Zhang, S.: Significant Difference in Hydrogen Isotope Composition Between Xylem and Tissue Water in Populus Euphratica, Plant Cell Environ., 39, 1848–1857, https://doi.org/10.1111/pce.12753, 2016.