the Creative Commons Attribution 4.0 License.
the Creative Commons Attribution 4.0 License.
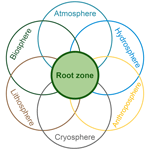
Root zone in the Earth system
Markus Hrachowitz
Lan Wang-Erlandsson
Fabrizio Fenicia
Qiaojuan Xi
Jianyang Xia
Wei Shao
Ge Sun
Hubert H. G. Savenije
The root zone is a vital part of the Earth system and a key element in hydrology, ecology, agronomy, and land surface processes. However, its definition varies across disciplines, creating barriers to interdisciplinary understanding. Moreover, characterizing the root zone is challenging due to a lack of consensus on definitions, estimation methods, and their merits and limitations. This opinion paper provides a holistic definition of the root zone from a hydrology perspective, including its moisture storage, deficit, and storage capacity. We demonstrate that the root zone plays a critical role in the biosphere, pedosphere, rhizosphere, lithosphere, atmosphere, and cryosphere of the Earth system. We underscore the limitations of the traditional reductionist approach in modelling this complex and dynamic zone and advocate for a shift towards a holistic, ecosystem-centred approach. We argue that a holistic approach offers a more systematic, simple, dynamic, scalable, and observable way to describe and predict the role of the root zone in Earth system science.
- Article
(5971 KB) - Full-text XML
- BibTeX
- EndNote
Plant roots developed before leaves during the Devonian Period, 416 to 360 million years ago (Kenrick and Strullu-Derrien, 2014). The development of root systems was a critical biological innovation that enabled land plants to spread and colonize the continental interior. Before this development, plants were limited to areas immediately adjacent to bodies of water due to their simple rhizoid-based rooting systems (Smart et al., 2023). Roots acted as pioneers of biological activity, representing a definitive line between sterile sediments and living soils. Fossil evidence indicates that deeper soils and larger plants appeared almost simultaneously (Kenrick and Strullu-Derrien, 2014).
There is a positive feedback between soils and plants, mediated by roots. Larger plants generally require more stable and deeper soils, while plant roots contribute to soil formation. The rapid expansion of terrestrial plants was largely enabled by newly developed rooting systems. Roots promoted physical, chemical, and biological rock weathering and increased terrestrial photosynthesis capability (Maeght et al., 2013), which significantly reduced atmospheric CO2. This reduction likely triggered the ice age and mass extinction in the late Devonian (Smart et al., 2023).
Thus, the evolution of plant root systems not only influenced the local soil microenvironment and individual plant life cycles but also affected the weathering of the lithosphere, the formation of the pedosphere, and atmospheric compositions. The initial appearance of rooting systems transformed the entire biosphere and the abiotic environment on the planet (Hetherington, 2019).
In recent decades, the root zone has received increasing attention from various disciplines, including hydrology, ecology, agronomy, biology, soil physics, atmospheric science, and landscape engineering. Despite this growing interest, there remains a lack of synthesis on the basic concept of the root zone, especially within the context of Earth system science. The root zone is arguably the least understood portion of the ecosystem that controls land surface processes (P. Wang et al., 2018; Y. Wang et al., 2018). Therefore, there is an urgent need to clarify its definition and to bridge the knowledge gaps between traditional disciplines.
This study has the following objectives:
-
Provide a definition of the root zone.
-
Propose a perspective of the root zone as a living, evolving, adapting, and essential part of the Earth system.
-
Advocate for a shift from the traditional reductionist approach towards a holistic, ecosystem-centred perspective of root zone hydrology in Earth system science.
2.1 Root zone
The root zone is the upper part of the subsurface that supports vegetation rooting (Sprenger et al., 2019), where water, air, and nutrients are available to sustain plants (see Fig. 1, and Rodríguez-Iturbe and Porporato, 2004). It typically extends through the unsaturated soil or parts thereof and may also reach the groundwater (Fan et al., 2017) or penetrate weathered bedrock (McCormick et al., 2021). The root zone is a hydrologically active layer, replenished with water during wet periods and supplying ecosystems with water to survive droughts in dry periods. It controls the partitioning of precipitation into infiltration, soil evaporation, plant transpiration, percolation to groundwater, and runoff (including surface runoff and subsurface storm flow) (e.g. Guswa, 2010; Lazarovitch et al., 2018).
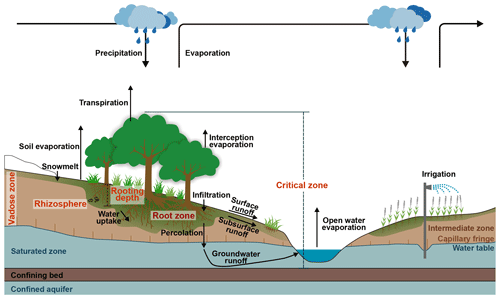
Figure 1Schematic diagram of the root zone in terrestrial hydrological processes and atmospheric moisture cycling, showing the difference between root zone and other similar terms, such as vadose zone, rooting depth, rhizosphere, and critical zone.
The root zone is a cross-scale concept, applicable at various scales, including the following (Delcourt and Delcourt, 1988; Blöschl and Sivapalan, 1995):
-
small scale (1–106 m2, plot, landscape, hillslope, and sub-catchment scales)
-
meso-scale (106–1010 m2, catchment scale)
-
large scale (above 1010 m2, river basin and continental scales).
2.2 Root zone water storage and root zone water deficit
The root zone acts as a buffer, ensuring continuous access to water for an ecosystem to bridge dry periods. Its shape, hydrological gradients, and internal water fluxes and processes are highly dynamic and complex. The interaction of plant roots, soil particles, bedrock fissures, water, nutrients, and micro-organisms creates an intricate system (McDonnell et al., 2007). From an ecosystem-centred hydrology perspective, the complex root zone can, reflecting a “Darwinian” approach (Harman and Troch, 2014), be viewed as a dynamic system within a living organism (Savenije and Hrachowitz, 2017; Savenije, 2024).
Water storage in the root zone can be conceptualized as a volume of water per unit area, represented as an average depth (e.g. in mm) (see Figs. 1 and 4). The water deficit (Sd) is defined as the difference between the maximum water volume and the actual root zone water storage at any time S(t). Water deficit and water storage are two sides of the same coin. During rainfall events, the root zone wets up and refills as water is retained. Once the root zone moisture exceeds its water retention capacity, excess water drains off by recharge to the groundwater (triggering groundwater flow) or by lateral preferential flow or as overland flow (by saturation excess or infiltration excess) directly to the stream. These processes may occur at the same time (McDonnell, 2013). In dry periods, ecosystems rely on the water stored in the root zone, leading to an increasing water deficit.
2.3 Root zone water storage capacity (SRmax)
The root zone storage capacity (SRmax) is the maximum volume of water stored in the subsurface that can be accessed by roots and that the root zone can contain to allow plants to overcome critical drought periods (Rodríguez-Iturbe and Porporato, 2004; Gao et al., 2014a; Klos et al., 2018; Stocker et al., 2023). SRmax is the core property of terrestrial hydrological systems and therefore also a key parameter in process-based hydrological models, determining the partitioning between drainage and evaporative fluxes. From a soil hydraulic perspective, SRmax represents the water volume that is stored in the subsurface between permanent wilting point and field capacity and that is within reach of roots. In other words, SRmax is equivalent to the maximum water deficit in the root zone, which defines the volumetric extent of the root zone (Gao et al., 2014a; Lapides et al., 2024). This concept can be likened to the storage capacity of an artificial reservoir, which is dimensioned to balance societal costs and benefits in light of an acceptable risk (i.e. the design return period). Similarly to human reservoir design, ecosystems show evidence of optimizing the root zone, balancing guaranteed water access (at a certain return period) against carbon expenditures for root growth and maintenance (Kleidon and Heiman, 1998; Guswa, 2008; Schymanski et al., 2008; Gao et al., 2014a).
2.4 Root zone and “similar” concepts
2.4.1 Root zone vs. vadose zone
The vadose zone (also known as the unsaturated zone) is the area underneath the land surface which forms the transition zone to the capillary fringe above the groundwater (Fig. 1). The root zone is contained within the unsaturated zone but is generally smaller. Below the root zone, the transition zone acts as a conduit facilitating percolation from the root zone to the groundwater, with very limited phase changes of water (i.e. evaporation) and without directly affecting the water balance at the land surface. In other words, the water percolating into the transition zone from above will eventually recharge the groundwater (with some temporal delay depending on the depth of the groundwater and soil types) and finally contribute to river flow. Deep confined groundwater, which is minimally connected to surface processes, generally does not factor into surface runoff calculations (Fitts, 2002). Consequently, the root zone, functioning as the active layer that is located mostly within the vadose zone, plays a pivotal role in catchment hydrology, largely determining how catchments respond to precipitation events.
2.4.2 Root zone vs. critical zone
The root zone, a key area where plants have evolved to adapt to their environment over time, constitutes the foundation of terrestrial ecosystems. As such, it is a vital component of the critical life zone on Earth (Banwart et al., 2017; Brantley et al., 2017). However, the definition of the critical zone remains a topic of debate. The most commonly accepted definition comes from the National Research Council in the US (NRC, 2001): the critical zone is the near-surface layer of the Earth, encompassing vegetation, soil, water, and rocks, all of which are essential for sustaining life. According to this definition, the critical zone extends “from the canopy top to the base of the groundwater zone” (Fig. 1). While there is widespread agreement on the upper boundary of the critical zone – the top of the vegetation canopy – the lower boundary is less defined and varies. For instance, some definitions cite the base of the groundwater zone (NRC, 2001), the bottom of the weathering zone (Guo and Lin, 2016), the base of active groundwater (Fan et al., 2016), or the storage of fresh groundwater (less than 50 years old) (Gleeson et al., 2016). Regardless of the specific version, the root zone is always included in critical-zone definitions. Furthermore, the root zone is the most dynamic layer within the critical zone, connecting the canopy to groundwater and encompassing all components of the critical zone: water, vegetation, soil, air, and bedrock.
2.4.3 Root zone vs. rhizosphere
The rhizosphere refers to the zone around roots where micro-organisms play crucial roles in biological processes essential for plant growth and health (Hiltner, 1904; Hartmann et al., 2008). It encompasses the roots themselves, the surrounding soil, and the narrow space between them, typically ranging from 1 to 4 mm (Kuzyakov and Razavi, 2019) (see Figs. 1 and 4). There are similarities between the rhizosphere and the root zone. Both are dynamic and alive. However, the rhizosphere specifically refers to the soil volume immediately surrounding living roots, extending only a few millimetres from the root surface. It is primarily studied to understand direct interactions with micro-organisms and to assess nutrient depletion zones. In contrast, the root zone concept is applied in larger-scale hydrology, agronomy, and land surface studies to evaluate the total water and nutrient resources available to plants. This results in a significant difference in volume, spanning 2 to 3 orders of magnitude between the rhizosphere and the root zone. The rhizosphere is more aligned with biological and soil science on a micro-scale (Kuzyakov and Razavi, 2019), whereas the root zone is better suited for broader ecological and hydrological investigations within Earth systems.
Earth system science is an interdisciplinary field geared toward the description of the Earth's structure and operations as a complex, integrated system (Steffen et al., 2020). Originating from early studies on interactions between the biosphere and geosphere and influenced by concepts such as the Gaia hypothesis (Lovelock, 1979), Earth System science gained prominence in the 1980s in response to the need for a comprehensive “science of the Earth”. In the latest conceptual model (Fig. 3 in Steffen et al., 2020), the root zone assumes a central role as the interface between roots, soils, vegetation, the lithosphere, the hydrosphere, the biosphere, the cryosphere, the atmosphere, and human society's production and consumption (Fig. 2). Despite its thin layer and the fact that it contains only 0.13 % of Earth's total freshwater (McCartney et al., 2024), the root zone profoundly influences land surface hydrology, land–atmospheric moisture exchange, and biogeochemical processes (Zehe et al., 2019).
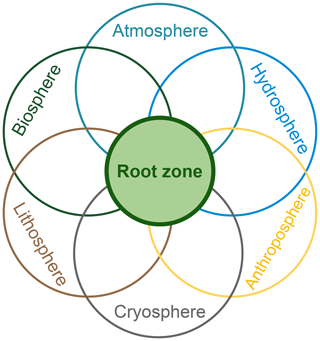
Figure 2Root zone is the interface between the hydrosphere, biosphere, lithosphere, atmosphere, cryosphere, and anthroposphere in the Earth system.
3.1 Roots and the hydrosphere
The terrestrial water cycle begins with precipitation, which is initially intercepted by vegetation and ground cover. From there, it either returns to the atmosphere through interception evaporation, runs off to drainage systems as overland flows, or infiltrates into the soil, eventually percolating into the root zone. Once infiltrated, water can be stored for plant use through transpiration; percolate deeper to recharge groundwater; or flow laterally, through preferential pathways, to the stream. Transpiration rates are regulated by plant characteristics, atmospheric water demand, and the availability of moisture in the root zone.
Overall, these processes – evaporation, transpiration, runoff, groundwater recharge – are largely controlled by the moisture content in the root zone (Rodríguez-Iturbe and Porporato, 2004). Therefore, root zone moisture plays a critical role in determining how precipitation is partitioned among these different pathways and affects the overall storage dynamics of the root zone.
In the global water balance over extended periods, precipitation is partitioned between runoff and evaporation, with the root zone playing a crucial role in this distribution. According to ERA-5 reanalysis data, the average annual terrestrial precipitation is 745 mm yr−1, of which 295 mm yr−1 (40 % of precipitation) returns to the oceans as streamflow, while 440 mm yr−1 (60 % of precipitation) is evaporated back into the atmosphere by ecosystems (Gao et al., 2023a).
The largest terrestrial water flux to the atmosphere is through vegetation root water uptake and, hence transpiration, which accounts for 60 %–90 % of total terrestrial evaporation globally (Jasechko et al., 2013; Schlesinger and Jasechko, 2014; Coenders-Gerrits et al., 2014; Wang et al., 2014; Lian et al., 2018). Despite global average canopy interception having a storage capacity of only around 2 mm (compared to approximately 200 mm SRmax; see Gao et al., 2023a), interception plays a significant role in total forest evaporation due to its continuous activity in all rainfall events (Gerrits et al., 2010; Sun et al., 2016).
In well-vegetated regions, soil evaporation is of minor relevance due to the protective effect of the canopy and litter layer but also due to the lack of turbulent exchange with depth (Brutsaert, 2014). Conversely, in sparsely vegetated areas such as deserts, soil evaporation can be more substantial. Globally, soil evaporation contributes approximately 6 % of total evaporation, with its significance being more prominent in arid than in humid regions (Wang-Erlandsson et al., 2014; Good et al., 2015; Zhang et al., 2017).
Plant roots, along with microbiotic and macrobiotic life, profoundly influence soil hydraulic properties and water cycle dynamics by creating soil macropores and adding organic matter (Ponge, 2015). These macropores, formed through the presence of living or decaying roots but also by animal activity enhanced by the presence of vegetation, act as large cracks or voids that reinforce infiltration and facilitate rapid water drainage as preferential flow pathways. This capability reduces the duration of soil saturation, thereby mitigating conditions that could lead to root rot.
Furthermore, organic matter from decomposed roots contributes to cementation within soils, altering their internal structure. Soils rich in organic matter typically exhibit higher porosity and water-holding capacity, crucial for retaining water in soil pores – a vital adaptation for terrestrial ecosystems in water-scarce environments.
The expansion of terrestrial ecosystems not only transforms hydrological processes within the root zone but also exerts significant impacts on the larger-scale water cycle (see Fig. 1 and Sect. 4.4). Modelling studies indicate that terrestrial ecosystems enhance terrestrial precipitation by 40 % (Xue et al., 2010). Comparisons between a green planet (fully forest-covered) and a desert planet (without vegetation) reveal that evaporation on continents triples and precipitation doubles in the former scenario (Fraedrich et al., 1999; Kleidon et al., 2000). These findings underscore the profound influence of root zone development in intensifying the global water cycle.
3.2 Roots and the biosphere
Roots are indispensable organs enabling plants to thrive and reproduce across diverse habitats. They serve crucial functions such as absorbing water and nutrients, which are essential for plant growth and performance. Additionally, roots anchor and stabilize plants in the soil; store chemical energy produced via photosynthesis in leaves in the form of carbohydrates and thus as biomass (Shekhar et al., 2019); and play a pivotal role in sensing local resources like water, nutrients, and phosphate. This sensing ability allows plants to efficiently access resources that are often heterogeneously distributed in the substrate's complex network of pores and fissures.
The root zone represents the product of long-term co-evolution between the biosphere and its inorganic environment, shaped by climatic and geological factors within the Earth system (Huggett, 2023). Similarly to the extensive global aboveground vegetation, the root zone likely spans a vast land area of approximately 103.9 ×106 km2 (70 % of total land area) (Ding et al., 2020).
Cutting-edge biological research has unveiled the root zone as a well-connected underground network of mycorrhizal fungi, akin to a nervous system, through which resources and information are exchanged among plants (Simard, 2018). This underscores that plants and their roots are integral components of a cohesive ecosystem rather than isolated entities. Further exploration of these interconnected relationships will be expanded upon in Sect. 4.1.5.
3.3 Roots and the lithosphere
From a long-term evolutionary perspective, roots play pivotal roles in promoting rock weathering and soil formation. Roots possess the ability to sense and navigate through heterogeneous structures, circumventing obstacles at both microstructural (volumes less than mm3) and macrostructural scales (Wang et al., 2020). They also act as “wedges”, physically opening up substructures (Pawlik et al., 2016) to access water stored in the bedrock (McCormick et al., 2021), while root-associated fungi further contribute to rock weathering and the enlargement of fractures (Schenk, 2008).
In the short term, roots exert a stabilizing effect by anchoring soil, which reduces erosion rates, enhances slope stability, and mitigates risks of landslides and debris flows (Vannoppen et al., 2017). Forest communities are particularly effective in minimizing erosional processes and supporting stability along hillslopes and riverbanks (Pawlik et al., 2016). Furthermore, roots significantly enhance soil strength, lowering the likelihood of shallow landslides (Cohen and Schwarz, 2017). The reduction in sediment flow facilitated by roots also yields basin-scale impacts on downstream river geomorphology (Ielpi et al., 2022; Cao et al., 2023).
3.4 Roots and the atmosphere
The root zone plays a pivotal role in land surface and climate modelling, a fact widely acknowledged in scientific literature (Milly and Dunne, 1994). The moisture status within the root zone critically influences water, energy, and carbon exchange fluxes between the land surface and the atmosphere, primarily through root water uptake and transpiration, as well as the associated latent heat flux, which enhances atmospheric water vapour. Transpiration, as a component of total land evaporation, depends on the balance between atmospheric water demand and the moisture supply in the subsurface and that which is accessible to plant roots. Vegetation and land cover thus actively regulate the exchange of water, energy, and carbon between the root zone and the atmosphere.
The root zone is not only influenced by but also actively influences the Earth's climate and the large-scale water cycle (Jackson et al., 1996). Firstly, evaporation entails a combined water and energy flux. From that perspective, incorporating accurate root zone data has significantly improved simulations of the land surface energy budget, particularly the latent heat flux (Zeng et al., 1998; Zheng and Wang, 2007). Secondly, compared to soil evaporation and canopy interception, the root zone of vegetation can access the deeper subsurface to extract water for transpiration. By supplying vapour to the atmosphere, the root zone is therefore a major component required to sustain atmospheric moisture levels and the associated downwind precipitation, which shapes the long-term climate pattern over considerable distances (van der Ent et al., 2010; van der Ent and Savenije, 2011; Wang-Erlandsson et al., 2018). Approximately 56 % of transpiration is expected to return to land as precipitation (Fig. 1, and van der Ent et al., 2014). The root zone helps transpiration to be sustained during dry periods, plays a particularly important role in dry-season length, and buffers against variability in precipitation (e.g. Keys et al., 2016; O'Connor et al., 2021).
3.5 Roots and the cryosphere
The connection between the root zone and the cryosphere is particularly pronounced in regions where vegetation covers frozen ground. In permafrost areas, the active layer undergoes seasonal freeze–thaw cycles, defining the maximum depth of thawing. Roots are constrained to grow within this active layer – the uppermost soil layer that thaws annually – because the permanent frozen layer below it and the ice layer are impermeable to root penetration (Blume-Werry et al., 2019).
In cold climates, the effects of snowmelt on the inflow to the root zone and soil freeze–thaw processes are crucial considerations (Gao et al., 2020, 2022; Wang-Erlandsson et al., 2016; Zhao et al., 2016; Dralle et al., 2021). Climate change exacerbates these dynamics by causing permafrost thawing, which expands the active layer and lowers the groundwater table. This thawing process opens up previously inaccessible soil volumes for potential root growth. The expansion of the active layer and changes in the groundwater table profoundly impact rooting depth and distribution in permafrost regions.
The dynamics of the active layer and variations in root zone storage are intricately intertwined in permafrost regions, influencing soil evaporation, plant transpiration, hydrologic connectivity, and runoff generation (Sugimoto et al., 2002; Suzuki et al., 2021). Understanding these interactions is essential for predicting how climate change will affect terrestrial ecosystems and hydrological processes in cold regions.
4.1 Reductionist perspective: processes within the root zone
From a reductionist perspective, the enormous heterogeneity and complexity of root zone features are characterized and catalogued by hydrologists, ecologists, pedologists, and microbiologists (McDonnell et al., 2007; Lin, 2010; Gao et al., 2018; Kuzyakov and Razavi, 2019). In this section, we outline the complexities within the root zone across five key categories: soil heterogeneities, rooting-distribution heterogeneity, flux heterogeneities, the plant's belowground zone of influence, the rhizosphere, and mycorrhizal fungi.
4.1.1 Soil heterogeneity
Soil heterogeneity exhibits variability in both horizontal and vertical dimensions, characterized by distinct horizons with diverse properties such as texture (varying proportions of sand, clay, and slit), mineral composition, and organic content. Soil also possesses varied hydraulic characteristics including porosity, moisture retention capacity, wilting point, and plant-available moisture. It consists of a complex mixture of minerals, organic matter, living organisms, gases, and water, earning it recognition as “the most complicated biomaterial on the planet” (NRC, 2009; Lin, 2010).
Despite its strong connection to soil, the root zone does not necessarily align with soil depth (Fig. 3) (Hahm et al., 2024). In many cases, particularly in regions with deep soils, the root zone is confined to the uppermost active layer of topsoil where the majority of hydrological and biogeochemical processes occur, even though the soil may extend much deeper. In water-stressed environments (Evaristo and McDonnell, 2017; Wang et al., 2022) or in regions with shallow groundwater (Fan et al., 2017), roots can reach the groundwater, expanding the definition of the root zone to include parts of the groundwater (see Singh et al., 2020). The depth to bedrock serves as the lower boundary of the soil, defining its overall depth. However, in karsts and other regions with shallow soil, roots can penetrate bedrock fissures to access water despite the challenging substrate conditions (McCormick et al., 2021; Lapides et al., 2024).
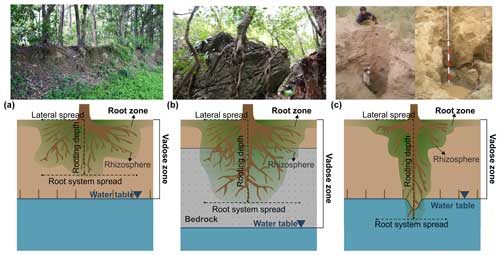
Figure 3Root zone is different from soil depth. Panel (a) is the root zone in a moderate climate. The root zone is the upper area of soil. (b) Root zone contains not only soil but also bedrock. (c) Root zone contains unsaturated zone and groundwater, for example, the phreatophytic roots in arid regions (P. Wang et al., 2018).
4.1.2 Rooting-distribution heterogeneity
The root distribution pattern in the soil profile plays a crucial role in determining how effectively plants can access water and nutrients. These patterns encompass the vertical and lateral spread of both coarse and fine roots, as well as the overall root density across the three-dimensional soil profile. Rooting depth, a key trait widely utilized in Earth system modelling, specifically refers to the depth to which roots extend vertically. However, it is important to note that root distribution does not always directly correlate with rooting depth (Zheng and Wang, 2007). For instance, an ecosystem dominated by deep-rooted plants with low root density may have a smaller overall root zone and, thus, a smaller accessible water volume compared to one with shallow-rooted plants that have a higher root density (Singh et al., 2020; van Oorschot et al., 2021).
Despite the critical importance of root distribution and concerted ongoing research efforts that compile an impressive richness of observations (e.g. Schenk and Jackson, 2005; Tumber-Davila et al., 2022), upscaling root distributions remains challenging. This is due to the fact that, although observations are available for several thousands of individual plants globally, these are essentially point- or plot-scale observations for which spatial covariance fields remain largely unknown. It is worth emphasizing that, even with detailed data on root distributions for individual plants, determining the precise extent of the root zone is challenging due to the complex network of pores, the fissures in soils and bedrock, the intricate hydrodynamic gradients, and the mycorrhizal fungi network (e.g. Casper et al., 2003; see Fig. 4).
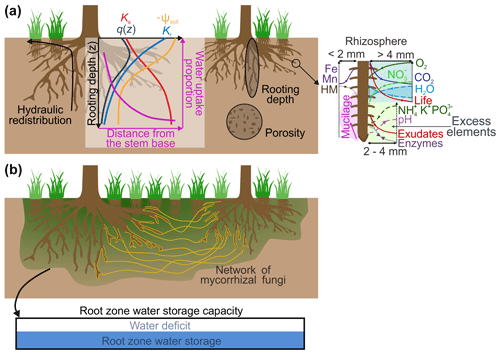
Figure 4Comparison of reductionist (a) and holistic (b) views of root zone and root zone water storage capacity. (a) The reductionist perspective estimates root zone storage capacity and transpiration based on rooting depth and soil properties, assuming a static view primarily relying on soil information. Root water uptake as a function of depth (q(z)) is shown for a soil profile with a dry upper layer (leading to more negative soil water potential (ψsoil) and low soil hydraulic conductivity (Ks)) and a deeper soil layer with higher water content (leading to less negative ψsoil and higher Ks). At the whole-plant scale, root conductivity (Kr) is correlated with the root surface area, which decreases exponentially with depth. The proportion of plants that took up water as a function of distance from the stem base is shown on the right side of the graph; this decreases with distance (adapted from Casper et al., 2003; Tumber-Davila et al., 2022; Bachofen et al., 2024). The top-right figure shows the generalization of rhizosphere extents and gradient types for the most investigated parameters: gases, root exudates, nutrients and excess elements, pH and Eh, enzyme activities, and microorganisms (from Kuzyakov and Razavi, 2019). (b) The holistic perspective views root zone storage as a volume per unit area, emphasizing an average depth. It estimates root zone capacity and transpiration based on plant water demand, reflecting a dynamic view influenced by climate and plant adaptation. The mycorrhizal fungi network enables the root zone to respond to environmental changes as a unified system.
4.1.3 Flux heterogeneity: preferential flow
Traditionally, in hydrology, the infiltration process was believed to be primarily controlled by matrix flow assuming that soil texture dictates hydraulic properties and infiltration capacity (Beven, 2021). However, mounting evidence now indicates that preferential flow routes rapidly transmit free water through the subsurface, dominating water movement across various scales (Uhlenbrook, 2006; Liu et al., 2012; Beven and Germann, 2013). In water-limited environments, the rooting systems of plants strongly influence infiltration capacity, velocity, and depth (Schenk and Jackson, 2002; Schenk, 2008). Infiltration replenishes moisture in the root zone through preferential pathways, while excess water either runs off or percolates into groundwater.
Recent research highlights the crucial role of mucilage exuded from roots and rhizosphere bacteria in forming liquid bridges at the root–soil interface. These bridges significantly impact the mechanical stability and hydraulic properties within the root zone (Bengough, 2012). The complexity introduced by preferential flow channels and their influence on hydraulic properties challenges the traditional soil-centric Darcy–Richards framework (Beven, 2012; Gao et al., 2023b).
4.1.4 Plant's belowground zone of influence
The concept of a plant's belowground zone of influence refers to how roots absorb water and nutrients not from a fixed area but from a highly dynamic and irregular zone (Casper et al., 2003). This zone exhibits complex and variable hydraulic and nutrient gradients between roots and their surroundings. From a reductionist, root-centred, micro-scale perspective, the belowground zone of influence quantifies how the influence of roots decreases with distance from the stem. The hydrological processes and shapes of root zones are extremely variable, plastic, irregular, and dynamic, making it effectively impossible to quantify or model this zone accurately.
However, viewing the root zone as a holistic system reveals emergent behaviours and spatial patterns that enable us to move beyond the complexities of gradient-based models and the heterogeneity of the plant's belowground zone of influence (see Fig. 4).
4.1.5 Rhizosphere and mycorrhizal fungi
The rhizosphere, as the dynamic area at the plant root–soil interface, is characterized by a multitude of biogeochemical processes driven by physical activities, such as water and nutrient dynamics (Fig. 4a and Bengough, 2012; Daly et al., 2017). The rhizosphere supports microbial growth (e.g. populations of bacteria ranging from 107 to 1012 per gram of rhizosphere soil), thereby stimulating biochemical processes that are crucial for plant nutrition and health (Hinsinger et al., 2009). Moreover, the mucilages and exudates of plants' roots, forming as soil aggregates, significantly alter the rhizosphere's hydraulic properties, such as infiltration capacity, water-holding capacity, and preferential flow (Daly et al., 2017).
Mycorrhizal fungi form a complex network of roots and fungal hyphae, often referred to as the “wood-wide web” (Beiler et al., 2009). These fungi create an underground continuous network that connects an estimated 90 % of land plant species (Bonfante and Genre, 2010), facilitating the exchange of resources such as carbon, water, and nutrients, as well as information about environmental conditions and threats such as insect infestations (Bonfante and Genre, 2010).
The existence of mycorrhizal fungi dramatically increases the root zone water and nutrient absorption capacity. The fine roots are usually thicker than 0.2 mm (Strand et al., 2008; Taylor et al., 2013), which means roots can only grow in macropores. Thus, roots have limited absorption capacity and can only use 4 %–7 % of the available soil volume (Guo et al., 2008). However, one spoon of soil can have mycorrhizal fungi as long as 1 km and as thin as 2–10 µm (Allen, 2007), which allows mycorrhizal fungi to grow in micropores among fine minerals which are unreachable for roots. These fungi enhance plant water uptake, leading it to be 7 times higher than without mycorrhizal fungi (Zhang et al., 2018), which is particularly beneficial during periods of water stress (Augé, 2001; Püschel et al., 2020); this is done by promoting water availability and transportation.
The intricate network of mycorrhizal fungi functions similarly to neural tissue in animals, allowing the root zone to respond to environmental changes – such as climate variations, water availability, nutrient levels, and threats – in a holistic and predictable manner.
4.2 Reductionists' root zone models
Although the hydrology community has advocated for moving beyond heterogeneities for some time (McDonnell et al., 2007), reductionist modelling remains prevalent in describing the root zone across hydrological models, dynamic vegetation models (DGVMs), and land surface schemes in Earth system models. Rooting depth varies among plant functional types (PFTs) and is, in these models, typically treated as a fixed parameter using lookup tables. Soil water-holding capacity, determined in laboratories, defines the amount of water retained under gravitational free drainage (van Oorschot et al., 2021). Soil depth, ranging widely from 1 m to over 10 m or until bedrock, is a critical parameter (Hidy et al., 2022; Wiltshire et al., 2021). Soil profiles are segmented into layers, varying from 3 to more than 10 (Seneviratne et al., 2010). The maximum storage capacity of the root zone, SRmax, combines rooting depth and soil water-holding capacity derived from soil texture data. In advanced models such as LPJ-GUESS, root distribution follows an exponential function constrained by maximum rooting depth, groundwater levels, and nutrient distribution (Smith et al., 2014). Furthermore, DGVMs integrate dynamic root modules to simulate root growth, architecture, and distribution in response to soil water and nitrogen availability (e.g. Lu et al., 2019).
However, we argue that this traditional perspective of the root zone, which assumes that the whole is the sum of its parts (Fig. 4a), is subject to several issues. First, the intricate network of pores and fissures in the substrate, extending both laterally and in depth, with complex gradients of water and nutrients, cannot be accurately simulated by mechanically combining rooting depth and soil properties (Fig. 4a). Second, this approach lacks detailed observations of root density and of vertical and lateral distributions, leading to significant uncertainties related to either the use of strong assumptions that may not by supported by data or a heavy reliance on parameter calibration. Third, the method overlooks fractal patterns in soil structure, pore networks, root morphology, and preferential flow paths. These fractal patterns embody organized complexity or simplicity that could enhance the realism of models, but they are typically broken up in reductionist approaches. Fourth, the root zone exhibits gradients in chemical, biological, and physical properties that vary radially and longitudinally along roots (McNear, 2013; Kuzyakov and Razavi, 2019). For instance, hydraulic redistribution is crucial for trees, redistributing water vertically from deeper, wetter soil layers to shallower, drier layers during the nighttime (Fig. 4a) (Bleby et al., 2010; Nadezhdina et al., 2010) but also laterally between individual plants (Hafner et al., 2021). Fifth, recent studies of the rhizosphere highlight the extraordinary complexity of underground root systems (e.g. Daly et al., 2017), which are difficult to adequately describe by means of reductionist methods. However, there is evidence that, from these small-scale heterogeneities, relatively stable and simple patterns emerge on larger scales. For example, small-scale studies of mycorrhizal fungi demonstrate that the root zone system responds as a whole to changes, providing a microscale foundation for holistic approaches to root zone modelling (Fig. 4).
5.1 Holistic perspective in hydrology
Holistic perspectives regard the root zone as an integrated living system shaped by fractal patterns, influenced by long-term self-organization and co-evolution. In contrast, reductionist models focus on determining how deep the root zone is. Holistic approaches instead ask broader questions regarding the size of the root zone and how much moisture it can buffer. Viewing the root zone holistically allows for more predictable behaviour, enabling simulation with simpler models, based on widely and readily available data.
5.1.1 Holistic root zone approach
The root zone, which is located beneath the surface and is not directly observable, can be characterized using an inverse modelling approach to infer its systematic behaviour. Various parameterizations driven by land surface water and energy fluxes, such as precipitation, evaporation, radiation, and biomass observations like net or gross primary production (Kleidon, 2004), play crucial roles in this process.
The mass curve technique (MCT), also referred to elsewhere as the memory method (van Oorschot et al., 2021, 2024), serves as an inverse model that employs a water-deficit approach based on observed land surface water budgets to estimate root zone processes (see Fig. 5) (Gao et al., 2014a; Wang-Erlandsson et al., 2016; Kühn et al., 2022). This holistic method allows hydrologists and ecologists to derive SRmax from ecosystem-scale observations of liquid water inflow (Fin) – i.e. the sum of precipitation (de Boer-Euser et al., 2016), snowmelt (Dralle et al., 2021), and irrigation (van Oorschot et al., 2024) – and water outflow (Fout, evaporation). Time series data of inflow and outflow are utilized to infer cumulative water deficits during dry periods (Eqs. 3 and 4). The largest cumulative deficit observed over a specific return period can be considered to be representative of the actual SRmax (Eq. 5).
is the water deficit on day tn+1, and is the accumulative water deficit on day tn+1.
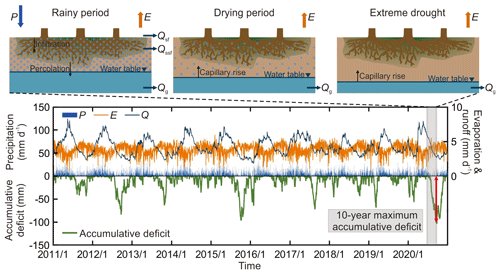
Figure 5The dynamic change in water deficit in the root zone from the wet season to the dry season. In the upper panel, the conceptual illustration of the water deficit change in root zone is shown with its effect on hydrology. P and E represent precipitation and evaporation, respectively. Qsf, Qssf, and Qg represent surface, subsurface, and groundwater runoff, respectively. The lower panel shows the precipitation (P), evaporation (E), runoff (Q), and accumulative water deficit in the root zone from 2011 to 2020. Note that the real-world root zone storage capacity cannot be smaller than the value SRmax estimated with the above method as, otherwise, there would not have been sufficient water available and accessible for vegetation to sustain the observed evaporation (Fout). SRmax is, therefore, in any case, a minimum and thus a lower limit to the root zone storage capacity. In principle, it can be argued that SRmax could be larger than that. However, increasing evidence from studies that estimate SRmax with optimality approaches (e.g. Kleidon, 2004; Guswa, 2008; Schymanksi et al., 2008) or as a model calibration parameter (e.g. Gao et al., 2014b; Nijzink et al., 2016; Hrachowitz et al., 2021; Wang et al., 2024) suggests that it is not likely that the real-world root zone storage capacity is larger than the SRmax associated with an ecosystem-specific dry spell return period. Instead, the results of these studies suggest that vegetation optimizes its below- and above-surface resource allocation to allow sufficiently large root systems to access water (and nutrients) in dry periods while also allowing sufficient above-surface growth to allow survival in the competition for light and for increased strength against windfall. In other words, the root system and, thus, SRmax are as large as necessary but not larger than that.
The holistic perspective requires integrated measurements (Reichstein et al., 2014), incorporating methods such as lysimeters and eddy covariance for determining land surface fluxes, satellite remote sensing, and catchment-scale water balance assessments (Gao et al., 2014a; Wang-Erlandsson et al., 2016; Kühn et al., 2022; Stocker et al., 2023). Lysimeters are particularly valuable tools for quantifying the water balance in the root zone, offering unique insights, especially in comparisons between vegetated and non-vegetated systems, highlighting the influence of vegetation dynamics on the water cycle (Seneviratne et al., 2012; Scanlon et al., 2005).
Satellite remote sensing provides extensive spatial and temporal data on land surface water fluxes, including precipitation and evaporation, which are crucial for deriving root zone processes. Catchment-scale water balance studies encompass variables such as precipitation and streamflow, offering valuable insights into long-term average evaporation patterns. Additionally, SRmax serves as a key parameter in conceptual hydrological models, often calibrated based on catchment precipitation and streamflow data (Gao et al., 2014b; Liang et al., 2024; Wang et al., 2024).
5.1.2 Climate and topography controls SRmax
The holistic perspective in modelling frameworks can be traced back to the bucket model used in the first global climate model by Manabe (1969). In this early study, SRmax was globally set at 150 mm, providing a reasonable estimation of the global average (Wang-Erlandsson et al., 2016; Gao et al., 2023a; Stocker et al., 2023). However, SRmax varies significantly across different climate zones and ecosystems.
In tropical rainforests (see Fig. 6), where rainfall is abundant throughout the year, SRmax tends to be small because water availability is not a limiting factor, allowing forests to develop tall canopies to compete for light. In temperate climates, where rainfall starts to constrain growth, ecosystems typically allocate more resources underground, enhancing their root zone capacity. In savannas, characterized by strong precipitation seasonality, ecosystems further expand their root zones to cope with prolonged droughts, often at the expense of aboveground biomass. Grasslands, on the other hand, may enter dormancy during droughts, exhibiting very limited SRmax (Fig. 6).
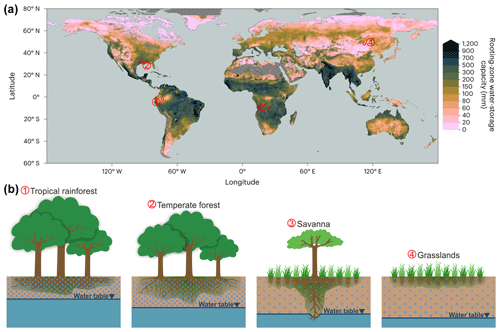
Figure 6(a) The global root zone storage capacity (from Stocker et al., 2023). (b) The conceptual illustration of aboveground biomass and root zone in different climate zones.
Interestingly, research has shown that ecosystems optimally adjust their root systems to their specific environments (Schymanski et al., 2008; Guswa, 2010), adapting to drought periods that vary from 5- to 40-year return periods depending on the ecosystem type (evergreen, deciduous, grassland, etc.) (Gao et al., 2014a; Wang-Erlandsson et al., 2016).
At the landscape scale, the spatial distribution of SRmax is largely influenced by topography (Fan et al., 2017; Gao et al., 2019). Topography also plays a critical role in determining runoff generation mechanisms, such as saturated overland flow in wetlands, subsurface storm flow in hillslopes, and infiltration excess overland flow on terraces (Savenije, 2010; Gharari et al., 2014).
5.1.3 Holistic root zone impacts on groundwater
The influence of the root zone on catchment hydrology is prominently observed through its impact on groundwater dynamics and baseflow recession. The root zone plays a crucial role in controlling water percolation to groundwater (Collenteur et al., 2021). Groundwater recharge and streamflow generation are closely linked as runoff is typically derived from groundwater under most conditions (Ali et al., 2011). Additionally, the root zone influences evaporation and plant transpiration from groundwater sources. Surface soil and root zone drying are moderated by upward capillary flow from the subsurface, sustaining evaporation during dry periods while reducing groundwater recharge over longer timescales.
A well-documented phenomenon is the diurnal cycle of streamflow and groundwater table fluctuations, particularly notable in arid and semi-arid catchments (Lundquist and Cayan, 2002; Wang and Pozdniakov, 2014; Yue et al., 2016). Vegetation, particularly in riparian areas, directly accesses groundwater for transpiration. Daytime root water uptake leads to declines in water tables, whereas water tables recover at night when transpiration rates diminish (Yue et al., 2016). This illustrates the direct hydraulic connection between root water uptake, groundwater dynamics, and river streamflow.
5.2 Holistic root zone in hydrology modelling
The concept of thresholds and hydrologic connectivity associated with runoff processes is crucial for understanding hydrological responses at both hillslope and catchment scales (Saffarpour et al., 2016), with the root zone playing a pivotal role as an integrated system. It is widely observed that runoff generation does not occur until the root zone reaches a critical moisture threshold, known as field capacity (Tromp-van Meerveld and McDonnell, 2006). Once this threshold is surpassed, the outflow pathways are activated, leading to runoff generation. This mechanism is often referred to as “fill and spill” (McDonnell et al., 2021) or “store and pour” (Phillips, 2022).
At the landscape scale, topography emerges as a primary factor influencing the mechanisms of runoff generation. Due to landscape heterogeneity (including topography and vegetation cover), the SRmax exhibits spatial variability. In many cases, SRmax increases with the elevation above the nearest drainage (HAND) (Fan et al., 2017). Consequently, saturation and runoff generation do not occur uniformly across an entire catchment (Hewlett and Hibbert, 1967; Arnbroise, 2004; Beven, 2012; Gao et al., 2019). Typically, during the onset of wetting seasons, riparian zones near the drainage experience the critical threshold first, initiating saturation excess flow. As precipitation and root zone moisture increase, the contributing areas expand, a phenomenon known as the theory of variable contribution area (Arnbroise, 2004). Accounting for this spatial heterogeneity of the root zone significantly improves the performance of runoff simulations (Gao et al., 2019).
These conceptual models are widely applied in global hydrological prediction practices, albeit with varied parameterizations and terminologies (e.g. Zhao, 1992; Perrin et al., 2003; Clark et al., 2008; Beven, 2012; Fenicia et al., 2014; Gao et al., 2019). In land surface and global hydrology studies comparing reductionist and holistic approaches, it is noteworthy that, despite its simplicity, the holistic method often outperforms more complex reductionist approaches in runoff simulation (Mao and Liu, 2019; Wang et al., 2021), particularly in ungauged basins (Hrachowitz et al., 2013). Recent studies highlight the potential of using climate-controlled root zone parameters to enhance water flux simulations in land surface models such as HTESSEL (Hydrology Tiled ECMWF Scheme for Surface Exchanges over Land), resulting in improved discharge simulation correlations across different regions (van Oorschot et al., 2021).
Moreover, the holistic approach to the root zone is gaining traction in studies of water quality, solute transport, and transit times, presenting a promising new framework for integrating material transport through the entire system (Harman and Xu Fei, 2024).
6.1 The impacts of climate change
Climate exerts significant control over root zone dynamics. The root zone plays a critical role in determining ecosystem resilience to droughts and climate variability, alongside other factors such as shifts in species composition and physiological adjustments like leaf shape and stomatal regulation (Zhang et al., 2023). Root growth is highly dynamic and responsive to environmental changes, with rapid carbon transfer from leaves to roots and soil organisms occurring within hours to days across different ecosystems (Melkikh and Sutormina, 2022).
The spatial variability of SRmax, reflecting climatic conditions, has been well documented (Kleidon and Heimann, 1998; Gao et al., 2014a; Wang-Erlandsson et al., 2016; Stocker et al., 2023), suggesting that temporal variations in SRmax are also plausible under changing climate regimes. Local factors such as topography and groundwater dynamics further influence root zone dynamics, with observations indicating rapid root growth towards declining water tables in desert phreatophyte vegetation (Orellana et al., 2012; P. Wang et al., 2018; Kuzyakov and Razavi, 2019).
At the basin scale, the temporal evolution of SRmax in response to climatic variability across multiple decades has been reported by several studies (Bouaziz et al., 2022; Tempel et al., 2024; Wang et al., 2024). Globally, analyses based on ERA-5 reanalysis data indicate an 11 % increase in global average SRmax over the past 4 decades (1982–2020), driven by intensifying drought conditions (Xi et al., 2024). This widespread increase in SRmax has been validated through dynamic identifiability analysis (DYNIA) algorithms using observed hydrometeorological data from US catchments (Liang et al., 2024).
6.2 The impacts of land use and land management activities
6.2.1 Agriculture
Agricultural activities exert a significant influence on the root zone, impacting various aspects that are crucial for food production and food security (Eisenhauer et al., 2024). Practices such as irrigation, fertilization, tilling, non-point source pollution, and salinization predominantly occur within the root zone, shaping its characteristics and functionality.
Ploughing, a fundamental agricultural practice, plays a pivotal role in altering and determining root zone depth. Over time, intensive cultivation can lead to the formation of a plough pan, typically situated 15 to 30 cm below the soil surface in clay-rich soils. This compacted layer acts as a barrier beneath the root zone, limiting water percolation and restricting root growth to the relatively shallow soil layer above it (Li et al., 2019). In such scenarios, the moisture-holding capacity of the topsoil becomes critical in defining the root zone storage capacity.
In irrigation agriculture, where additional water is supplied to the root zone during dry periods, the SRmax often becomes smaller compared to under natural conditions with similar climates (Xi et al., 2021; Hauser et al., 2022; van Oorschot et al., 2024). Studies indicate that, due to irrigation practices (excluding considerations of climate change impacts), rooting depth can be reduced by approximately 5 %, equivalent to an approximate loss of 8 cm in depth or about 11 600 km3 of rooted volume (Hauser et al., 2022). At a global scale, research has shown a consistent decrease in SRmax in croplands when irrigation practices are factored in as an additional water supply (van Oorschot et al., 2024).
6.2.2 Deforestation and afforestation
Deforestation can significantly reduce SRmax (Hrachowitz et al., 2021). Recovery of SRmax following deforestation can take more than a decade to restore to previous levels (Nijzink et al., 2016). Conversely, regions experiencing woody encroachment often see root depths deepening by approximately 38 cm compared to the previous dominant vegetation (Hauser et al., 2022).
The root zone's role extends to evaluating the outcomes of large-scale ecological initiatives. For example, projects like China's Grain for Green Programme and the Three-North Shelterbelt Forest Program have proven to be beneficial for soil conservation, flood control, biodiversity enhancement, and carbon sequestration (Chen et al., 2019; Li et al., 2021). However, these initiatives have also increased water consumption through evaporation, posing challenges for water management in arid regions where societal water demands compete with ecosystem services (Feng et al., 2016). The underground root zone is the key piece in the puzzle with regard to connecting the isolated dots and explaining all these ecological and hydrological phenomena in one framework.
Transitioning from traditional blue-water management to integrated water resources management involves incorporating both blue- and green-water considerations – surface and groundwater – into a unified framework (Falkenmark, 2000). Root zone moisture plays a pivotal role in linking land surface fluxes, water resources, soil erosion, and carbon sequestration, thereby influencing the success and sustainability of such ambitious ecological projects (Sun et al., 2021).
6.2.3 Urbanization
Urbanization has profound effects on the root zone, transforming it from a permeable to an impermeable structure, which leads to increased storm water runoff and reduced vegetation evaporation, as noted by Hao et al. (2018). Furthermore, urbanization significantly impacts the underground mycorrhizal fungi network, altering the hydrological processes within the root zone and catchment areas, as well as influencing the land surface energy budget. These changes contribute to intensified urban inundation and exacerbate the urban-heat-island effect (Fletcher et al., 2013).
There is growing interest in leveraging vegetation to manage hydrology in urban environments, exemplified by initiatives like China's “Sponge City” initiative (Xia et al., 2017). The root zone acts as a natural sponge in larger natural landscapes such as forests and grasslands, while green roofs and urban gardens serve as smaller-scale sponges within cities and neighbourhoods. Increasing the SRmax has the potential to enhance flood prevention, improve water purification, mitigate the urban-heat-island effect, and enhance the aesthetic value of urban areas (Palmer et al., 2015).
7.1 Observations
Accurately measuring root traits and characteristics of the root zone is among the most challenging tasks in ecological, agricultural, and hydrological research, particularly in field studies. For instance, determining the rooting depth of grasses and crops typically involves soil core sampling, while estimating coarse tree roots relies on allometric equations, and fine tree roots are assessed using soil cores. Despite efforts such as the global rooting depth synthesis study covering 2200 observations worldwide (Fan et al., 2017) and others (Tumber-Davila et al., 2022), this remains a relatively small sample compared to the vast number of 3.04 trillion trees globally (Crowther et al., 2015), not to mention the numerous grasses and agricultural crops. Consequently, descriptions of the spatial distributions of root systems remain uncertain at scales from hillslopes to globally.
Remote sensing products such as SMAP and SMOS provide relatively high-resolution estimates of near-surface moisture, but their capabilities are confined to the top few centimetres of soil (Xu et al., 2021). Estimating root zone moisture from surface measurements involves specific assumptions and different types of models (Entekhabi et al., 2010; Reichle et al., 2019; Bouaziz et al., 2020; Kim et al., 2023). In Earth system models (ESMs), uncertainties in projections of dry-season water availability are considerable, sometimes exceeding 200 % of the ensemble mean, underscoring the need for more accurate observational data.
To deepen our quantitative descriptions of root zone hydrology, particularly from a holistic perspective, additional experiments and measurements across diverse climates and landscapes are crucial. Methods such as field control experiments, rhizobox studies (Nie et al., 2013; Zhou et al., 2022; Maan et al., 2023), and long-term lysimeter measurements (Scanlon et al., 2005) are essential. Interestingly, rhizobox experiments have shown that detailed root distribution information may not be necessary to accurately estimate water budgets (Maan et al., 2023). This prompts the following question: which variables should we prioritize when making observations to enhance our understanding of root zone processes?
7.2 Root zone biogeochemistry
In this opinion paper, our focus has been centred on root zone hydrology, recognizing water as the crucial link among Earth system spheres. We contend that the root zone, viewed holistically, holds broad implications for biogeochemistry studies, encompassing carbon, nitrogen, phosphate, pollutants, and microbial communities, thereby influencing Earth system science on a global scale. The burgeoning field of root microbiome research in ecology is poised to significantly deepen our understanding of rhizosphere biotic processes within the root zone, their ecosystem-wide importance, and their impacts on large-scale biogeochemical cycles (McNear, 2013).
The successful integration of the holistic root zone concept into hydrology and land surface models sets the stage for incorporating more biogeochemical processes, such as carbon and nutrient dynamics, as part of an integrated system. For instance, considering carbon dynamics, plants with roots play a pivotal role in the global carbon budget by sequestering CO2 through photosynthesis and releasing CO2 via respiration, influencing the climate system significantly (Bian et al., 2023). Root zone dynamics are crucial for carbon sequestration strategies, particularly in predicting plant responses to elevated CO2 levels under climate change scenarios (Nie et al., 2013; Bian et al., 2023). Among carbon storage alternatives, root zone carbon presents the highest uncertainty, yet it holds substantial potential for carbon neutrality and sequestration strategies, necessitating further experimental and modelling investigations (Friedlingstein et al., 2022).
The holistic modelling framework proves to be particularly adept at integrating biogeochemical processes, leveraging conceptual models as the primary approach to simulate fluxes of carbon, nitrogen, and phosphorus. This approach not only enhances our understanding of root zone processes but also contributes to advancing our capabilities in predicting and managing Earth system dynamics in response to global change (Violle et al., 2014; Reichstein et al., 2014).
7.3 Making models alive for future prediction
The call to integrate a more dynamic and “alive” root zone into Earth system models is urgent and critical for improving predictions across various disciplines (P. Wang et al., 2018; Y. Wang et al., 2018). Static root models have shown significant discrepancies in simulating land surface water and energy dynamics (Jing et al., 2014; Cai et al., 2018; Drewniak, 2019; Liu et al., 2020; Zheng and Wang, 2007), largely due to their inability to capture the adaptability of root zones to changing environmental conditions. Despite pioneering efforts to incorporate dynamic root behaviour into land surface and dynamic vegetation models (Y. Wang et al., 2018; Lu et al., 2019; Sakschewski et al., 2021), reductionist modelling remains dominant in Earth system models.
Currently, holistic root zone modelling primarily serves as a diagnostic approach. However, its potential lies in offering simpler and more realistic simulations. Moving forward, several approaches can enhance the integration of holistic perspectives into Earth system models:
-
Space-for-time exchange. Analysing spatial transitions in ecosystems can provide insights into ecohydrological strategies and can help predict hydrological responses under future climate scenarios. For instance, using methods like the mass curve technique (MCT), Singh et al. (2020) demonstrated how SRmax changes spatially with climate, offering a framework to forecast belowground and aboveground biomass variations.
-
Optimization approach. Leveraging optimality principles to integrate water, carbon, nutrient dynamics, and vegetation responses based on ecological optimality can enhance predictions of root dynamics and their impacts on environmental changes (Schymanski et al., 2008; P. Wang et al., 2018; Hunt et al., 2024).
-
Incorporating physical laws. Earth system modelling currently relies heavily on Newtonian laws of mass, energy, and momentum conservation, which is typical of reductionist approaches. However, broadening the scope to include additional physical laws, such as evolutionary theory and the second law of thermodynamics, can reduce parameter calibration needs and constrain model uncertainties (Savenije, 2024).
These approaches collectively aim to advance our understanding of and prediction capabilities with regard to Earth system dynamics, emphasizing the need for more integrated and comprehensive models that capture the dynamic interactions within the root zone and beyond.
7.4 Planetary stewardship
The root zone, as a critical interface between the natural geosphere and anthroposphere (Fig. 3 in Steffen et al., 2020), holds profound implications for planetary stewardship and sustainable development. Human activities like agriculture, urbanization, deforestation and afforestation, land use change, and the use of pesticides and fertilization profoundly impact the root zone. Understanding the root zone is pivotal for managing green-water footprints, integrated water resources, and carbon sequestration (Wang-Erlandsson et al., 2022). It serves a crucial role in dividing precipitation into green water (used by terrestrial ecosystems) and blue water (available for human use), thereby influencing Earth system resilience (Falkenmark, 2000).
The management of the root zone is essential for sustaining both green and blue water within safe planetary boundaries, which is crucial for achieving global sustainability development goals (SDGs) such as SDG2 (zero hunger), SDG6 (clean water and sanitation), SDG11 (sustainable cities and communities), SDG13 (climate action), and SDG15 (life on land). Proper management practices are, therefore, indispensable with regard to maintaining Earth system resilience and achieving these SDGs (Stewart-Koster et al., 2023; Wang-Erlandsson et al., 2022).
Integrating the impacts of human activities on the root zone into Earth system models (ESMs) is crucial for advancing scientific understanding, informed decision-making, and effective management of the root zone. This integration will enhance our ability to predict and mitigate the consequences of human actions on water resources, ecosystems, and global climate dynamics.
The root zone is the crucial element linking multiple spheres of the Earth surface system, including the hydrosphere, biosphere, lithosphere, atmosphere, cryosphere, and anthroposphere. Although many disciplines are studying the root zone from different angles, this has not yet been done in a systematic way. This study explored the differences and linkages between the root zone and other similar terminologies, such as the vadose zone, critical zone, rhizosphere, and rooting depth. For the root zone in Earth system studies, we underscored the heterogeneity within the root zone, including the complexities of soil, root distribution, preferential flow, plants' belowground zones of influence, the rhizosphere, and mycorrhizal fungi. However, viewing the root zone as an integrated living system, influenced by long-term self-organization and co-evolution, allows for emergent and predictable behaviour, enabling simulation with simpler models based on widely and readily available data. We advocate for a paradigm shift towards ecosystem-centred root zone studies in Earth system science in order to develop “living” models for more realistic future prediction, particularly in response to climate change and intensifying human activities in the Anthropocene.
No code or datasets were used in this article.
HG conceptualized the study and was responsible for writing the original draft. All authors provided valuable insights and feedback to enhance the manuscript and contributed to the editing and revision of the manuscript.
At least one of the (co-)authors is a member of the editorial board of Hydrology and Earth System Sciences. The peer-review process was guided by an independent editor, and the authors also have no other competing interests to declare.
Publisher's note: Copernicus Publications remains neutral with regard to jurisdictional claims made in the text, published maps, institutional affiliations, or any other geographical representation in this paper. While Copernicus Publications makes every effort to include appropriate place names, the final responsibility lies with the authors.
This research has been supported by the National Natural Science Foundation of China (grant nos. 42122002 and 42071081).
This research has been supported by the National Natural Science Foundation of China (grant nos. 42122002 and 42071081).
This paper was edited by Lixin Wang and reviewed by four anonymous referees.
Ali, G. A., L'Heureux, C., Roy, A. G., Turmel, M. C., and Courchesne, F.: Linking spatial patterns of perched groundwater storage and stormflow generation processes in a headwater forested catchment, Hydrol. Process., 25, 3843–3857, https://doi.org/10.1002/hyp.8238, 2011.
Allen, M. F.: Mycorrhizal fungi: Highways for water and nutrients in arid soils, Vadose Zone J., 6, 291–297, https://doi.org/10.2136/vzj2006.0068, 2007.
Arnbroise, B.: Variable, `active' versus `contributing' areas or periods: a necessary distinction, Hydrol. Process., 18, 1149–1155, https://doi.org/10.1002/hyp.5536, 2004.
Augé, R. M.: Water relations, drought and vesicular-arbuscular mycorrhizal symbiosis, Mycorrhiza, 11, 3–42, https://doi.org/10.1007/s005720100097, 2001.
Bachofen, C., Tumber-Davila, S. J., Mackay, D. S., McDowell, N. G., Carminati, A., Klein, T., Stocker, B. D., Mencuccini, M., and Grossiord, C.: Tree water uptake patterns across the globe, New Phytol., 242, 1891–1910, https://doi.org/10.1111/nph.19762, 2024.
Banwart, S. A., Bernasconi, S. M., Blum, W. E. H., de Souza, D. M., Chabaux, F., Duffy, C., Kercheva, M., Krám, P., Lair, G. J., Lundin, L., Menon, M., Nikolaidis, N. P., Novak, M., Panagos, P., Ragnarsdottir, K. V., Robinson, D. A., Rousseva, S., de Ruiter, P., van Gaans, P., Weng, L., White, T., and Zhang, B.: Soil Functions in Earth's Critical Zone: Key Results and Conclusions, Adv. Agron., 142, 1–27, https://doi.org/10.1016/bs.agron.2016.11.001, 2017.
Beiler, K. J., Durall, D. M., Simard, S. W., Maxwell, S. A., and Kretzer, A. M.: Architecture of the wood-wide web: Rhizopogon spp. genets link multiple Douglas-fir cohorts, New Phytol., 185, 543–553, https://doi.org/10.1111/j.1469-8137.2009.03069.x, 2009.
Bengough, A. G.: Water Dynamics of the Root Zone: Rhizosphere Biophysics and Its Control on Soil Hydrology, Vadose Zo. J., 11, vzj2011.0111, https://doi.org/10.2136/vzj2011.0111, 2012.
Beven, K.: The era of infiltration, Hydrol. Earth Syst. Sci., 25, 851–866, https://doi.org/10.5194/hess-25-851-2021, 2021.
Beven, K. and Germann, P.: Macropores and water flow in soils revisited, Water Resour. Res., 49, 3071–3092, https://doi.org/10.1002/wrcr.20156, 2013.
Beven, K. J.: Rainfall-runoff modeling: the primer. Rainfall-runoff Model, Primer, 15, 84–96, 2012.
Bian, C., Xia, J., Zhang, X., Huang, K., Cui, E., Zhou, J., Wei, N., Wang, Y. P., Lombardozzi, D., Goll, D. S., Knauer, J., Arora, V., Yuan, W., Sitch, S., Friedlingstein, P., and Luo, Y.: Uncertainty and Emergent Constraints on Enhanced Ecosystem Carbon Stock by Land Greening, J. Adv. Model. Earth Syst., 15, e2022MS003397, https://doi.org/10.1029/2022MS003397, 2023.
Bleby, T. M., Mcelrone, A. J., and Jackson, R. B.: Water uptake and hydraulic redistribution across large woody root systems to 20 m depth, Plant Cell Environ., 33, 2132–2148, https://doi.org/10.1111/j.1365-3040.2010.02212.x, 2010.
Blöschl, G. and Sivapalan, M.: Scale issues in hydrological modelling: A review, Hydrol. Process., 9, 3–4, https://doi.org/10.1002/hyp.3360090305, 1995.
Blume-Werry, G., Milbau, A., Teuber, L. M., Johansson, M., and Dorrepaal, E.: Dwelling in the deep–strongly increased root growth and rooting depth enhance plant interactions with thawing permafrost soil, New Phytol., 223, 1328–1339, https://doi.org/10.1111/nph.15903, 2019.
Bonfante, P. and Genre, A.: Mechanisms underlying beneficial plant-fungus interactions in mycorrhizal symbiosis, Nat. Commun., 1, 48, https://doi.org/10.1038/ncomms1046, 2010.
Bouaziz, L. J. E., Steele-Dunne, S. C., Schellekens, J., Weerts, A. H., Stam, J., Sprokkereef, E., Winsemius, H. H. C., Savenije, H. H. G., and Hrachowitz, M.: Improved Understanding of the Link Between Catchment-Scale Vegetation Accessible Storage and Satellite-Derived Soil Water Index, Water Resour. Res., 56, e2019WR026365, https://doi.org/10.1029/2019WR026365, 2020.
Bouaziz, L. J. E., Aalbers, E. E., Weerts, A. H., Hegnauer, M., Buiteveld, H., Lammersen, R., Stam, J., Sprokkereef, E., Savenije, H. H. G., and Hrachowitz, M.: Ecosystem adaptation to climate change: The sensitivity of hydrological predictions to time-dynamic model parameters, Hydrol. Earth Syst. Sci., 26, 1295–1318, https://doi.org/10.5194/hess-26-1295-2022, 2022.
Brantley, S. L., Eissenstat, D. M., Marshall, J. A., Godsey, S. E., Balogh-Brunstad, Z., Karwan, D. L., Papuga, S. A., Roering, J., Dawson, T. E., Evaristo, J., Chadwick, O., McDonnell, J. J., and Weathers, K. C.: Reviews and syntheses: on the roles trees play in building and plumbing the critical zone, Biogeosciences, 14, 5115–5142, https://doi.org/10.5194/bg-14-5115-2017, 2017.
Brutsaert, W.: Daily evaporation from drying soil: Universal parameterization with similarity, Water Resour. Res., 50, 3206–3215, https://doi.org/10.1002/2013WR014872, 2014.
Cai, G., Vanderborght, J., Langensiepen, M., Schnepf, A., Hüging, H., and Vereecken, H.: Root growth, water uptake, and sap flow of winter wheat in response to different soil water conditions, Hydrol. Earth Syst. Sci., 22, 2449–2470, https://doi.org/10.5194/hess-22-2449-2018, 2018.
Cao, Z., Duan, H., Ma, R., Shen, M., and Yang, H.: Remarkable effects of greening watershed on reducing suspended sediment flux in China's major rivers, Sci. Bull., 68, 2285–2288, https://doi.org/10.1016/j.scib.2023.08.036, 2023.
Casper, B. B., Schenk, H. J., and Jackson, R. B.: Defining a plant's belowground zone of influence, Ecology, 84, 2313–2321, https://doi.org/10.1890/02-0287, 2003.
Chen, C., Park, T., Wang, X., Piao, S., Xu, B., Chaturvedi, R. K., Fuchs, R., Brovkin, V., Ciais, P., Fensholt, R., Tømmervik, H., Bala, G., Zhu, Z., Nemani, R. R., and Myneni, R. B.: China and India lead in greening of the world through land-use management, Nat. Sustain., 2, 122–129, https://doi.org/10.1038/s41893-019-0220-7, 2019.
Clark, M. P., Slater, A. G., Rupp, D. E., Woods, R. A., Vrugt, J. A., Gupta, H. V., Wagener, T., and Hay, L. E.: Framework for Understanding Structural Errors (FUSE): A modular framework to diagnose differences between hydrological models, Water Resour. Res., 44, W00B02, https://doi.org/10.1029/2007WR006735, 2008.
Coenders-Gerrits, A. M. J., Van Der Ent, R. J., Bogaard, T. A., Wang-Erlandsson, L., Hrachowitz, M., and Savenije, H. H. G.: Uncertainties in transpiration estimates, Nature, 506, E1–E2, https://doi.org/10.1038/nature12925, 2014.
Cohen, D. and Schwarz, M.: Tree-root control of shallow landslides, Earth Surf. Dynam., 5, 451–477, https://doi.org/10.5194/esurf-5-451-2017, 2017.
Collenteur, R. A., Bakker, M., Klammler, G., and Birk, S.: Estimation of groundwater recharge from groundwater levels using nonlinear transfer function noise models and comparison to lysimeter data, Hydrol. Earth Syst. Sci., 25, 2931–2949, https://doi.org/10.5194/hess-25-2931-2021, 2021.
Crowther, T. W., Glick, H. B., Covey, K. R., Bettigole, C., Maynard, D. S., Thomas, S. M., Smith, J. R., Hintler, G., Duguid, M. C., Amatulli, G., Tuanmu, M. N., Jetz, W., Salas, C., Stam, C., Piotto, D., Tavani, R., Green, S., Bruce, G., Williams, S. J., Wiser, S. K., Huber, M. O., Hengeveld, G. M., Nabuurs, G. J., Tikhonova, E., Borchardt, P., Li, C. F., Powrie, L. W., Fischer, M., Hemp, A., Homeier, J., Cho, P., Vibrans, A. C., Umunay, P. M., Piao, S. L., Rowe, C. W., Ashton, M. S., Crane, P. R., and Bradford, M. A.: Mapping tree density at a global scale, Nature, 525, 201–205, https://doi.org/10.1038/nature14967, 2015.
Daly, K. R., Cooper, L. J., Koebernick, N., Evaristo, J., Keyes, S. D., van Veelen, A., and Roose, T.: Modelling water dynamics in the rhizosphere, Rhizosphere, 4, 139–151, https://doi.org/10.1016/j.rhisph.2017.10.004, 2017.
de Boer-Euser, T., McMillan, H. K., Hrachowitz, M., Winsemius, H. C., and Savenije, H. H. G.: Influence of soil and climate on root zone storage capacity, Water Resour. Res., 52, 2009–2024, https://doi.org/10.1002/2015WR018115, 2016.
Delcourt, H. and Delcourt, P.: Quaternary landscape ecology: Relevant scales in space and time, Landscape Ecol., 2, 23–44, https://doi.org/10.1007/BF00138906, 1988.
Ding, Z., Peng, J., Qiu, S., and Zhao, Y.: Nearly Half of Global Vegetated Area Experienced Inconsistent Vegetation Growth in Terms of Greenness, Cover, and Productivity, Earth Future, 8, e2020EF001618, https://doi.org/10.1029/2020EF001618, 2020.
Dralle, D. N., Hahm, W. J., Chadwick, K. D., McCormick, E., and Rempe, D. M.: Technical note: Accounting for snow in the estimation of root zone water storage capacity from precipitation and evapotranspiration fluxes, Hydrol. Earth Syst. Sci., 25, 2861–2867, https://doi.org/10.5194/hess-25-2861-2021, 2021.
Drewniak, B. A.: Simulating dynamic roots in the energy Exascale Earth system land model, J. Adv. Model. Earth Syst., 11, 338–359, https://doi.org/10.1029/2018MS001334, 2019.
Eisenhauer, N., Frank, K., Weigelt, A., Bartkowski, B., Beugnon, R., Liebal, K., Mahecha, M., Quaas, M., Al-Halbouni, D., Bastos, A., Bohn, F. J., de Brito, M. M., Denzler, J., Feilhauer, H., Fischer, R., Fritsche, I., Guimaraes-Steinicke, C., Hänsel, M., Haun, D. B. M., Herrmann, H., Huth, A., Kalesse-Los, H., Koetter, M., Kolleck, N., Krause, M., Kretschmer, M., Leitão, P. J., Masson, T., Mora, K., Müller, B., Peng, J., Pöhlker, M. L., Ratzke, L., Reichstein, M., Richter, S., Rüger, N., Sánchez-Parra, B., Shadaydeh, M., Sippel, S., Tegen, I., Thrän, D., Umlauft, J., Wendisch, M., Wolf, K., Wirth, C., Zacher, H., Zaehle, S., and Quaas, J.: A belowground perspective on the nexus between biodiversity change, climate change, and human well-being, J. Sustain. Agric. Environ., 3, e212108, https://doi.org/10.1002/sae2.12108, 2024.
Entekhabi, D., Njoku, E. G., O'Neill, P. E., Kellogg, K. H., Crow, W. T., Edelstein, W. N., Entin, J. K., Goodman, S. D., Jackson, T. J., Johnson, J., Kimball, J., Piepmeier, J. R., Koster, R. D., Martin, N., McDonald, K. C., Moghaddam, M., Moran, S., Reichle, R., Shi, J. C., Spencer, M. W., Thurman, S. W., Tsang, L., and Van Zyl, J.: The Soil Moisture Active Passive (SMAP) Mission, Proc. IEEE, 98, 704–716, https://doi.org/10.1109/JPROC.2010.2043918, 2010.
Evaristo, J. and McDonnell, J. J.: Prevalence and magnitude of groundwater use by vegetation: a global stable isotope meta-analysis, Sci. Rep., 7, 44110, https://doi.org/10.1038/srep44110, 2017.
Falkenmark, M.: Competing freshwater and ecological services in the river basin perspective: An expanded conceptual framework, Water Int., 25, 172–177, https://doi.org/10.1080/02508060008686815, 2000.
Fan, J., McConkey, B., Wang, H., and Janzen, H.: Root distribution by depth for temperate agricultural crops, Field Crop. Res., 189, 68–74, https://doi.org/10.1016/j.fcr.2016.02.013, 2016.
Fan, Y., Miguez-Macho, G., Jobbagy, E. G., Jackson, R. B., and Otero-Casal, C.: Hydrologic regulation of plant rooting depth, P. Natl. Acad. Sci. USA, 114, 10572–10577, https://doi.org/10.1073/pnas.1712381114, 2017.
Feng, X., Fu, B., Piao, S., Wang, S., Ciais, P., Zeng, Z., Lü, Y., Zeng, Y., Li, Y., Jiang, X., and Wu, B.: Revegetation in China's Loess Plateau is approaching sustainable water resource limits, Nat. Clim. Change, 6, 1019–1022, https://doi.org/10.1038/nclimate3092, 2016.
Fenicia, F., Kavetski, D., Savenije, H. H. G., Clark, M. P., Schoups, G., Pfister, L., and Freer, J.: Catchment properties, function, and conceptual model representation: is there a correspondence?, Hydrol. Process., 28, 2451–2467, https://doi.org/10.1002/hyp.9726, 2014.
Fitts, C. R.: Groundwater Science, in: 1st Edn., Elsevier, ISBN 978-0-08-049503-3, 2002.
Fletcher, T. D., Andrieu, H., and Hamel, P.: Understanding, management and modelling of urban hydrology and its consequences for receiving waters: A state of the art, Adv. Water Resour., 51, 261–279, https://doi.org/10.1016/j.advwatres.2012.09.001, 2013.
Fraedrich, K., Kleidon, A., and Lunkeit, F.: A green planet versus a desert world: Estimating the effect of vegetation extremes on the atmosphere, J. Climate, 12, 3156–3163, https://doi.org/10.1175/1520-0442(1999)012<3156:AGPVAD>2.0.CO;2, 1999.
Friedlingstein, P., Jones, M. W., O'Sullivan, M., Andrew, R. M., Bakker, D. C. E., Hauck, J., Le Quéré, C., Peters, G. P., Peters, W., Pongratz, J., Sitch, S., Canadell, J. G., Ciais, P., Jackson, R. B., Alin, S. R., Anthoni, P., Bates, N. R., Becker, M., Bellouin, N., Bopp, L., Chau, T. T. T., Chevallier, F., Chini, L. P., Cronin, M., Currie, K. I., Decharme, B., Djeutchouang, L. M., Dou, X., Evans, W., Feely, R. A., Feng, L., Gasser, T., Gilfillan, D., Gkritzalis, T., Grassi, G., Gregor, L., Gruber, N., Gürses, Ö., Harris, I., Houghton, R. A., Hurtt, G. C., Iida, Y., Ilyina, T., Luijkx, I. T., Jain, A., Jones, S. D., Kato, E., Kennedy, D., Goldewijk, K. K., Knauer, J., Korsbakken, J. I., Körtzinger, A., Landschützer, P., Lauvset, S. K., Lefèvre, N., Lienert, S., Liu, J., Marland, G., McGuire, P. C., Melton, J. R., Munro, D. R., Nabel, J. E. M. S., Nakaoka, S. I., Niwa, Y., Ono, T., Pierrot, D., Poulter, B., Rehder, G., Resplandy, L., Robertson, E., Rödenbeck, C., Rosan, T. M., Schwinger, J., Schwingshackl, C., Séférian, R., Sutton, A. J., Sweeney, C., Tanhua, T., Tans, P. P., Tian, H., Tilbrook, B., Tubiello, F., Van Der Werf, G. R., Vuichard, N., Wada, C., Wanninkhof, R., Watson, A. J., Willis, D., Wiltshire, A. J., Yuan, W., Yue, C., Yue, X., Zaehle, S., and Zeng, J.: Global Carbon Budget 2021, Earth Syst. Sci. Data, 14, 1917–2005, https://doi.org/10.5194/essd-14-1917-2022, 2022.
Gao, H., Hrachowitz, M., Schymanski, S. J., Fenicia, F., Sriwongsitanon, N., and Savenije, H. H. G.: Climate controls how ecosystems size the root zone storage capacity at catchment scale, Geophys. Res. Lett., 41, 7916–7923, https://doi.org/10.1002/2014GL061668, 2014a.
Gao, H., Hrachowitz, M., Fenicia, F., Gharari, S., and Savenije, H. H. G.: Testing the realism of a topography-driven model (FLEX-Topo) in the nested catchments of the Upper Heihe, China, Hydrol. Earth Syst. Sci., 18, 1895–1915, https://doi.org/10.5194/hess-18-1895-2014, 2014b.
Gao, H., Sabo, J.L, Chen, X., Liu, Z., Yang, Z., Ren, Z., and Liu, M.: Landscape heterogeneity and hydrological processes: a review of landscape-based hydrological models, Landscape Ecol., 33, 1461–1480, https://doi.org/10.1007/s10980-018-0690-4, 2018.
Gao, H., Birkel, C., Hrachowitz, M., Tetzlaff, D., Soulsby, C., and Savenije, H. H. G.: A simple topography-driven and calibration-free runoff generation module, Hydrol. Earth Syst. Sci., 23, 787–809, https://doi.org/10.5194/hess-23-787-2019, 2019.
Gao, H., Dong, J., Chen, X., Cai, H., Liu, Z., Jin, Z., Mao, D., Yang, Z., and Duan, Z.: Stepwise modeling and the importance of internal variables validation to test model realism in a data scarce glacier basin, J. Hydrol., 591, 125457, https://doi.org/10.1016/j.jhydrol.2020.125457, 2020.
Gao, H., Han, C., Chen, R., Feng, Z., Wang, K., Fenicia, F., and Savenije, H.: Frozen soil hydrological modeling for a mountainous catchment northeast of the Qinghai-Tibet Plateau, Hydrol. Earth Syst. Sci., 26, 4187–4208, https://doi.org/10.5194/hess-26-4187-2022, 2022.
Gao, H., Liu, J., Gao, G., and Xia, J.: Ecological and hydrological perspectives of the water retention concept, Acta Geogr. Sin., 78, 139–148, 2023a.
Gao, H., Fenicia, F., and Savenije, H. H. G.: HESS Opinions: Are soils overrated in hydrology?, Hydrol. Earth Syst. Sci., 27, 2607–2620, https://doi.org/10.5194/hess-27-2607-2023, 2023b.
Gerrits, A. M. J., Pfister, L., and Savenije, H. H. G.: Spatial and temporal variability of canopy and forest floor interception in a beech forest, Hydrol. Process., 24, 3011–3025, https://doi.org/10.1002/hyp.7712, 2010.
Gharari, S., Hrachowitz, M., Fenicia, F., Gao, H., and Savenije, H. H. G.: Using expert knowledge to increase realism in environmental system models can dramatically reduce the need for calibration, Hydrol. Earth Syst. Sci., 18, 4839–4859, https://doi.org/10.5194/hess-18-4839-2014, 2014.
Gleeson, T., Befus, K. M., Jasechko, S., Luijendijk, E., and Cardenas, M. B.: The global volume and distribution of modern groundwater, Nat. Geosci., 9, 161–164, https://doi.org/10.1038/ngeo2590, 2016.
Good, S. P., Noone, D., and Bowen, G.: Hydrologic connectivity constrains partitioning of global terrestrial water fluxes, Science, 349, 175–177, https://doi.org/10.1126/science.aaa5931, 2015.
Guo, D., Xia, M., Wei, X., Chang, W., Liu, Y., and Wang, Z.: Anatomical traits associated with absorption and mycorrhizal colonization are linked to root branch order in twenty-three Chinese temperate tree species, New Phytol., 180, 673–683, https://doi.org/10.1111/j.1469-8137.2008.02573.x, 2008.
Guo, L. and Lin, H.: Critical zone research and observatories: Current status and future perspectives, Vadose Zone J., 15, 1–14, https://doi.org/10.2136/vzj2016.06.0050, 2016.
Guswa, A. J.: The influence of climate on root depth: A carbon cost-benefit analysis, Water Resour. Res., 44, W02427, https://doi.org/10.1029/2007WR006384, 2008.
Guswa, A. J.: Effect of plant uptake strategy on the water-optimal root depth, Water Resour. Res., 46, W09601, https://doi.org/10.1029/2010WR009122, 2010.
Hafner, B. D., Hesse, B. D., and Grams, T. E. E.: Friendly neighbours: Hydraulic redistribution accounts for one quarter of water used by neighbouring drought stressed tree saplings, Plant Cell Environ., 44, 1243–1256, https://doi.org/10.1111/pce.13852, 2021.
Hahm, W. J., Dralle, D. N., Lapides, D. A., Ehlert, R. S., and Rempe, D. M.: Geologic Controls on Apparent Root-Zone Storage Capacity, Water Resour. Res., 60, e2023WR035362, https://doi.org/10.1029/2023WR035362, 2024.
Hao, L., Huang, X., Qin, M., Liu, Y., Li, W., and Sun, G.: Ecohydrological processes explain urban dry island effects in a wet region, southern China, Water Resour., 54, 6757–6771, https://doi.org/10.1029/2018WR023002, 2018.
Harman, C. and Troch, P. A.: What makes Darwinian hydrology “Darwinian”? Asking a different kind of question about landscapes, Hydrol. Earth Syst. Sci., 18, 417–433, https://doi.org/10.5194/hess-18-417-2014, 2014.
Harman, C. J. and Xu Fei, E.: mesas.py v1.0: a flexible Python package for modeling solute transport and transit times using StorAge Selection functions, Geosci. Model Dev., 17, 477–495, https://doi.org/10.5194/gmd-17-477-2024, 2024.
Hartmann, A., Rothballer, M., and Schmid, M.: Lorenz Hiltner, a pioneer in rhizosphere microbial ecology and soil bacteriology research, Plant Soil, 312, 7–14, https://doi.org/10.1007/s11104-007-9514-z, 2008.
Hauser, E., Sullivan, P. L., Flores, A. N., Hirmas, D., and Billings, S. A.: Global-Scale Shifts in Rooting Depths Due To Anthropocene Land Cover Changes Pose Unexamined Consequences for Critical Zone Functioning, Earth's Future, 10, e2022EF002897, https://doi.org/10.1029/2022EF002897, 2022.
Hetherington, A. J.: Evolution of Plant Rooting Systems, in: Encyclopedia of Life Sciences, John Wiley & Sons, Ltd., 1–10, https://doi.org/10.1002/9780470015902.a0028341, 2019.
Hewlett, J. D. and Hibbert, A. R. Factors affecting the response of small watersheds to precipitation in humid areas, in: Forest hydrology, edited by: Sopper, W. E. and Lull, H. W., Pergamon Press, New York, https://api.semanticscholar.org/CorpusID:128648858 (last access: 11 October 2024), 1967.
Hidy, D., Barcza, Z., Hollós, R., Dobor, L., Ács, T., Zacháry, D., Filep, T., Pásztor, L., Incze, D., Dencső, M., Tóth, E., Merganičová, K., Thornton, P., Running, S., and Fodor, N.: Soil-related developments of the Biome-BGCMuSo v6.2 terrestrial ecosystem model, Geosci. Model Dev., 15, 2157–2181, https://doi.org/10.5194/gmd-15-2157-2022, 2022.
Hiltner, L.: Ueber neuere Erfahrungen und Probleme auf dem Gebiete der Bodenbakteriologie und unter besonderer Berücksichtigung der Grundungung und Brache, Arb. Deut. Landw. Gesell, 98, 59–78, 1904.
Hinsinger, P., Bengough, A. G., Vetterlein, D., and Young, I. M.: Rhizosphere: Biophysics, biogeochemistry and ecological relevance, Plant Soil, 321, 117–152, https://doi.org/10.1007/s11104-008-9885-9, 2009.
Hrachowitz, M., Savenije, H. H. G., Blöschl, G., McDonnell, J. J., Sivapalan, M., Pomeroy, J. W., Arheimer, B., Blume, T., Clark, M. P., Ehret, U., Fenicia, F., Freer, J. E., Gelfan, A., Gupta, H. V., Hughes, D. A., Hut, R. W., Montanari, A., Pande, S., Tetzlaff, D., Troch, P. A., Uhlenbrook, S., Wagener, T., Winsemius, H. C., Woods, R. A., Zehe, E., and Cudennec, C.: A decade of Predictions in Ungauged Basins (PUB) – a review, Hydrolog. Sci. J., 58, 1198–1255, https://doi.org/10.1080/02626667.2013.803183, 2013.
Hrachowitz, M., Stockinger, M., Coenders-Gerrits, M., Van Der Ent, R., Bogena, H., Lücke, A., and Stumpp, C.: Reduction of vegetation-accessible water storage capacity after deforestation affects catchment travel time distributions and increases young water fractions in a headwater catchment, Hydrol. Earth Syst. Sci., 25, 4887–4915, https://doi.org/10.5194/hess-25-4887-2021, 2021.
Huggett, R.: Soil as part of the Earth system, Prog. Phys. Geogr., 47, 454–466, https://doi.org/10.1177/03091333221147655, 2023.
Hunt, A. G., Sahimi, M., Ghanbarian, B., and Poveda, G.: Predicting ecosystem net primary productivity by percolation theory and optimality principle, Water Resour. Res., 60, e2023WR036340, https://doi.org/10.1029/2023WR036340, 2024.
Ielpi, A., Lapôtre, M. G. A., Gibling, M. R., and Boyce, C. K.: The impact of vegetation on meandering rivers, Nat. Rev. Earth Environ., 3, 165–178, https://doi.org/10.1038/s43017-021-00249-6, 2022.
Jackson, R. B., Canadell, J., Ehleringer, J. R., Mooney, H. A., Sala, O. E., and Schulze, E. D.: A global analysis of root distributions for terrestrial biomes, Oecologia, 108, 389–411, https://doi.org/10.1007/BF00333714, 1996.
Jasechko, S., Sharp, Z. D., Gibson, J. J., Birks, S. J., Yi, Y., and Fawcett, P. J.: Terrestrial water fluxes dominated by transpiration, Nature, 496, 347–350, https://doi.org/10.1038/nature11983, 2013.
Jing, C. Q., Li, L., Chen, X., and Luo, G. P.: Comparison of root water uptake functions to simulate surface energy fluxes within a deep-rooted desert shrub ecosystem, Hydrol. Process., 28, 5436–5449, https://doi.org/10.1002/hyp.10047, 2014.
Kenrick, P. and Strullu-Derrien, C.: The origin and early evolution of roots, Plant Physiol., 166, 570–580, https://doi.org/10.1104/pp.114.244517, 2014.
Keys, P. W., Wang-Erlandsson, L., and Gordon, L. J.: Revealing Invisible Water: Moisture Recycling as an Ecosystem Service, PLoS One, 11, e0151993, https://doi.org/10.1371/journal.pone.0151993, 2016.
Kim, Y., Park, H., Kimball, J. S., Colliander, A., and McCabe, M. F.: Global estimates of daily evapotranspiration using SMAP surface and root-zone soil moisture, Remote Sens. Environ., 298, 113803, https://doi.org/10.1016/j.rse.2023.113803, 2023.
Kleidon, A.: Global datasets and rooting zone depth inferred from inverse methods, J. Climate, 17, 2714–2722, https://doi.org/10.1175/1520-0442(2004)017<2714:GDORZD>2.0.CO;2, 2004.
Kleidon, A. and Heimann, M.: A method of determining rooting depth from a terrestrial biosphere model and its impacts on the global water and carbon cycle, Global Change Biol., 4, 275–286, https://doi.org/10.1046/j.1365-2486.1998.00152.x, 1998.
Kleidon, A., Fraedrich, K., and Heimann, M.: A green planet versus a desert world: Estimating the maximum effect of vegetation on the land surface climate, Climatic Change, 44, 471–493, https://doi.org/10.1023/A:1005559518889, 2000.
Klos, P. Z., Goulden, M. L., Riebe, C. S., Tague, C. L., O'Geen, A. T., Flinchum, B. A., Safeeq, M., Conklin, M. H., Hart, S. C., Berhe, A. A., Hartsough, P. C., Holbrook, W. S., and Bales, R. C.: Subsurface plant-accessible water in mountain ecosystems with a Mediterranean climate, Wiley Interdisciplin. Rev.-Water, 5, e1277, https://doi.org/10.1002/wat2.1277, 2018.
Kühn, N., Spiegel, M. P., Tovar, C., Willis, K. J., and Macias-Fauria, M.: Seeing roots from space: aboveground fingerprints of root depth in vegetation sensitivity to climate in dry biomes, Environ. Res. Lett., 17, 114062, https://doi.org/10.1088/1748-9326/ac9d4f, 2022.
Kuzyakov, Y. and Razavi, B. S.: Rhizosphere size and shape: Temporal dynamics and spatial stationarity, Soil Biol. Biochem., 135, 343–360, https://doi.org/10.1016/j.soilbio.2019.05.011, 2019.
Lapides, D. A., Hahm, W. J., Forrest, M., Rempe, D. M., Hickler, T., and Dralle, D. N.: Inclusion of bedrock vadose zone in dynamic global vegetation models is key for simulating vegetation structure and function, Biogeosciences, 21, 1801–1826, https://doi.org/10.5194/bg-21-1801-2024, 2024.
Lazarovitch, N., Vanderborght, J., Jin, Y., and van Genuchten, M. T.: The Root Zone: Soil Physics and Beyond, Vadose Zone J., 17, 1–6, https://doi.org/10.2136/vzj2018.01.0002, 2018.
Li, C., Fu, B., Wang, S., Stringer, L. C., Wang, Y., Li, Z., Liu, Y., and Zhou, W.: Drivers and impacts of changes in China's drylands, Nat. Rev. Earth Environ., 2, 858–873, https://doi.org/10.1038/s43017-021-00226-z, 2021.
Li, Y., Zhai, Z., Cong, P., Zhang, Y., Pang, H., Dong, G., and Gao, J.: Effect of plough pan thickness on crop growth parameters, nitrogen uptake and greenhouse gas (CO2 and N2O) emissions in a wheat-maize double-crop rotation in the Northern China Plain: A one-year study, Agr. Water Manage., 213, 534–545, https://doi.org/10.1016/j.agwat.2018.10.044, 2019.
Lian, X., Piao, S., Huntingford, C., Li, Y., Zeng, Z., Wang, X., Ciais, P., McVicar, T. R., Peng, S., Ottlé, C., Yang, H., Yang, Y., Zhang, Y., and Wang, T.: Partitioning global land evapotranspiration using CMIP5 models constrained by observations, Nat. Clim. Change, 8, 640–646, https://doi.org/10.1038/s41558-018-0207-9, 2018.
Liang, J., Gao, H., Fenicia, F., Xi, Q., Wang, Y., and Savenije, H. H. G.: Widespread increase of root zone storage capacity in the United States, EGUsphere [preprint], https://doi.org/10.5194/egusphere-2024-550, 2024.
Lin, H.: Earth's Critical Zone and hydropedology: concepts, characteristics, and advances, Hydrol. Earth Syst. Sci., 14, 25–45, https://doi.org/10.5194/hess-14-25-2010, 2010.
Liu, D., Tian, F., Hu, H., and Hu, H.: Le rôle du ruissellement latéral dans l'écoulement de surface et les caractéristiques de la production d'écoulement dans le plateau de Loess, en Chine, Hydrolog. Sci. J., 57, 1107–1117, https://doi.org/10.1080/02626667.2012.695870, 2012.
Liu, X., Chen, F., Barlage, M., and Niyogi, D.: Implementing dynamic rooting depth for improved simulation of soil moisture and land surface feedbacks in Noah-MP-Crop, J. Adv. Model. Earth Syst., 12, e2019MS001786. https://doi.org/10.1029/2019MS001786, 2020.
Lovelock, J.: Gaia: A New Look at Life on Earth, Oxford Univ. Press, ISBN 9780198784883, 1979.
Lu, H., Yuan, W., and Chen, X.: A Processes-Based Dynamic Root Growth Model Integrated Into the Ecosystem Model, J. Adv. Model. Earth Syst., 11, 4614–4628, https://doi.org/10.1029/2019MS001846, 2019.
Lundquist, J. D. and Cayan, D. R.: Seasonal and spatial patterns in diurnal cycles in streamflow in the western United States, J. Hydrometeorol., 3, 591–603, https://doi.org/10.1175/1525-7541(2002)003<0591:SASPID>2.0.CO;2, 2002.
Maan, C., ten Veldhuis, M. C., and van de Wiel, B. J. H.: Dynamic root growth in response to depth-varying soil moisture availability: a rhizobox study, Hydrol. Earth Syst. Sci., 27, 2341–2355, https://doi.org/10.5194/hess-27-2341-2023, 2023.
Maeght, J. L., Rewald, B., and Pierret, A.: How to study deep roots-and why it matters, Front. Plant Sci., 4, 1–14, https://doi.org/10.3389/fpls.2013.00299, 2013.
Manabe, S.: Climate and the ocean circulation, Mon. Weather Rev., 97, 775–805, https://doi.org/10.1175/1520-0493(1969)097<0775:CATOC>2.3.CO;2, 1969.
Mao, G. and Liu, J.: WAYS v1: A hydrological model for root zone water storage simulation on a global scale, Geosci. Model Dev., 12, 5267–5289, https://doi.org/10.5194/gmd-12-5267-2019, 2019.
McCartney, M., Rex, W., Yu, W., Uhlenbrook, S., and von Gnechten, R.: Change in global freshwater storage, IWMI Working Paper 202, IWMI – International Water Management Institute, Colombo, Sri Lanka, https://doi.org/10.5337/2022.204, 2024.
McCormick, E. L., Dralle, D. N., Hahm, W. J., Tune, A. K., Schmidt, L. M., Chadwick, K. D., and Rempe, D. M.: Widespread woody plant use of water stored in bedrock, Nature, 597, 225–229, https://doi.org/10.1038/s41586-021-03761-3, 2021.
McDonnell, J. J.: Are all runoff processes the same?, Hydrol. Process., 27, 4103–4111, 2013.
McDonnell, J. J., Sivapalan, M., Vache, K., Dunn, S., Grant, G., Haggerty, R., Hinz, C., Hooper, R., Kirchner, J., Roderick, M. L., Selker, J., and Weiler, M.: Moving beyond heterogeneity and process complexity: A new vision for watershed hydrology, Water Resour. Res., 43, W07301, https://doi.org/10.1029/2006WR005467, 2007.
McDonnell, J. J., Spence, C., Karran, D. J., van Meerveld, H. J., and Harman, C. J.: Fill-and-Spill: A Process Description of Runoff Generation at the Scale of the Beholder, Water Resour. Res., 57, e2020WR027514, https://doi.org/10.1029/2020WR027514, 2021.
McNear, D.: The rhizosphere-roots, soil and everything in between, Nature Education Knowledge, 4, 1, http://www.nature.com/scitable/knowledge/library/the-rhizosphere-roots-soil-and-67500617 (last access: 11 October 2024), 2013.
Melkikh, A. V. and Sutormina, M. I.: From leaves to roots: Biophysical models of transport of substances in plants, Prog. Biophys. Mol. Biol., 169–170, 53–83, https://doi.org/10.1016/j.pbiomolbio.2022.01.002, 2022.
Milly, P. C. D. and Dunne, K. A.: Sensitivity of the global water cycle to the water-holding capacity of land, J. Climate, 7, 506–526, https://doi.org/10.1175/1520-0442(1994)007<0506:SOTGWC>2.0.CO;2, 1994.
Nadezhdina, N., David, T. S., David, J. S., Ferreira, M. I., Dohnal, M., Tesař, M., Gartner, K., Leitgeb, E., Nadezhdin, V., Cermak, J., Jimenez, M. S., and Morales, D.: Trees never rest: the multiple facets of hydraulic redistribution, Ecohydrology, 3, 431–444, https://doi.org/10.1002/eco.148, 2010.
Nie, M., Lu, M., Bell, J., Raut, S., and Pendall, E.: Altered root traits due to elevated CO2: A meta-analysis, Global Ecol. Biogeogr., 22, 1095–1105, https://doi.org/10.1111/geb.12062, 2013.
Nijzink, R., Hutton, C., Pechlivanidis, I., Capell, R., Arheimer, B., Freer, J., Han, D., Wagener, T., McGuire, K., Savenije, H., and Hrachowitz, M.: The evolution of root-zone moisture capacities after deforestation: A step towards hydrological predictions under change?, Hydrol. Earth Syst. Sci., 20, 4775–4799, https://doi.org/10.5194/hess-20-4775-2016, 2016.
NRC – National Research Council: Basic Research Opportunities in Earth Science, National Academies Press, Washington, D.C., USA, https://doi.org/10.17226/9981, 2001.
NRC – National Research Council: Science and Decisions: Advancing Risk Assessment, National Academies Press, Washington, D.C., USA, https://doi.org/10.17226/12209, 2009.
O'Connor, J. C., Dekker, S. C., Staal, A., Tuinenburg, O. A., Rebel, K. T., and Santos, M. J.: Forests buffer against variations in precipitation, Global Chang Biol., 27, 4686–4696, https://doi.org/10.1111/gcb.15763, 2021.
Orellana, F., Verma, P., Loheide, S. P., and Daly, E.: Monitoring and modeling water-vegetation interactions in groundwater-dependent ecosystems, Rev. Geophys., 50, RG3003, https://doi.org/10.1029/2011RG000383, 2012.
Palmer, M. A., Liu, J., Matthews, J. H., Mumba, M., and D'Odorico, P.: Manage water in a green way, Science, 349, 584–585, https://doi.org/10.1126/science.aac7778, 2015.
Pawlik, Ł., Phillips, J. D., and Šamonil, P.: Roots, rock, and regolith: Biomechanical and biochemical weathering by trees and its impact on hillslopes – A critical literature review, Earth-Sci. Rev., 159, 142–159, https://doi.org/10.1016/j.earscirev.2016.06.002, 2016.
Perrin, C., Michel, C., and Andréassian, V.: Improvement of a parsimonious model for streamflow simulation, J. Hydrol., 279, 275–289, https://doi.org/10.1016/S0022-1694(03)00225-7, 2003.
Phillips, J. D.: Store and pour: Evolution of flow systems in landscapes, Catena, 216, 106357, https://doi.org/10.1016/j.catena.2022.106357, 2022.
Ponge, J. F.: The soil as an ecosystem, Biol. Fert. Soils, 51, 645–648, https://doi.org/10.1007/s00374-015-1016-1, 2015.
Püschel, D., Bitterlich, M., Rydlová, J., and Jansa, J.: Facilitation of plant water uptake by an arbuscular mycorrhizal fungus: a Gordian knot of roots and hyphae, Mycorrhiza, 30, 299–313, https://doi.org/10.1007/s00572-020-00949-9, 2020.
Reichle, R. H., Liu, Q., Koster, R. D., Crow, W., De Lannoy, G. J. M., Kimball, J. S., Ardizzone, J. V., Bosch, D., Colliander, A., Cosh, M., Kolassa, J., Mahanama, S. P., Prueger, J., Starks, P., and Walker, J. P.: Version 4 of the SMAP Level-4 Soil Moisture Algorithm and Data Product, J. Adv. Model. Earth Syst., 11, 3106–3130, https://doi.org/10.1029/2019MS001729, 2019.
Reichstein, M., Bahn, M., Mahecha, M. D., Kattge, J., and Baldocchi, D. D.: Linking plant and ecosystem functional biogeography, P. Natl. Acad. Sci. USA, 111, 13697–13702, https://doi.org/10.1073/pnas.1216065111, 2014.
Rodriguez-Iturbe, I. and Porporato A.: Ecohydrology of Water Controlled Ecosystems: Soil Moisture and Plant Dynamics, Cambridge Univ. Press, New York, ISBN 0-521-819431, 2004.
Saffarpour, S., Western, A. W., Adams, R., and McDonnell, J. J.: Multiple runoff processes and multiple thresholds control agricultural runoff generation, Hydrol. Earth Syst. Sci., 20, 4525–4545, https://doi.org/10.5194/hess-20-4525-2016, 2016.
Sakschewski, B., von Bloh, W., Drüke, M., Sörensson, A. A., Ruscica, R., Langerwisch, F., Billing, M., Bereswill, S., Hirota, M., Oliveira, R. S., Heinke, J., and Thonicke, K.: Variable tree rooting strategies are key for modelling the distribution, productivity and evapotranspiration of tropical evergreen forests, Biogeosciences, 18, 4091–4116, https://doi.org/10.5194/bg-18-4091-2021, 2021.
Savenije, H. H. G.: HESS Opinions “Topography driven conceptual modelling (FLEX-Topo)”, Hydrol. Earth Syst. Sci., 14, 2681–2692, https://doi.org/10.5194/hess-14-2681-2010, 2010.
Savenije, H. H. G.: The hydrological system as a living organism, Proc. IAHS, 385, 1–4, https://doi.org/10.5194/piahs-385-1-2024, 2024.
Savenije, H. H. G. and Hrachowitz, M.: HESS Opinions “catchments as meta-organisms – A new blueprint for hydrological modelling”, Hydrol. Earth Syst. Sci., 21, 1107–1116, https://doi.org/10.5194/hess-21-1107-2017, 2017.
Scanlon, B. R., Levitt, D. G., Reedy, R. C., Keese, K. E., and Sully, M. J.: Ecological controls on water-cycle response to climate variability in deserts, P. Natl. Acad. Sci. USA, 102, 6033–6038, https://doi.org/10.1073/pnas.0408571102, 2005.
Schenk, H. J.: Soil depth, plant rooting strategies and species' niches, New Phytol., 178, 223–225, https://doi.org/10.1111/j.1469-8137.2008.02427.x, 2008.
Schenk, H. J. and Jackson, R. B.: The global biogeography of roots, Ecol. Monogr., 72, 311–328, https://doi.org/10.1890/0012-9615(2002)072[0311:TGBOR]2.0.CO;2, 2002.
Schenk, H. J. and Jackson, R. B.: Mapping the global distribution of deep roots in relation to climate and soil characteristics, Geoderma, 126, 129–140, https://doi.org/10.1016/j.geoderma.2004.11.018, 2005.
Schlesinger, W. H. and Jasechko, S.: Transpiration in the global water cycle, Agr. Forest Meteorol., 189–190, 115–117, https://doi.org/10.1016/j.agrformet.2014.01.011, 2014.
Schymanski, S. J., Sivapalan, M., Roderick, M. L., Beringer, J., and Hutley, L. B.: An optimality-based model of the coupled soil moisture and root dynamics, Hydrol. Earth Syst. Sci., 12, 913–932, https://doi.org/10.5194/hess-12-913-2008, 2008.
Seneviratne, S. I., Corti, T., Davin, E. L., Hirschi, M., Jaeger, E. B., Lehner, I., Orlowsky, B., and Teuling, A. J.: Investigating soil moisture-climate interactions in a changing climate: A review, Earth-Sci. Rev., 99, 125–161, https://doi.org/10.1016/j.earscirev.2010.02.004, 2010.
Seneviratne, S. I., Lehner, I., Gurtz, J., Teuling, A. J., Lang, H., Moser, U., Grebner, D., Menzel, L., and Schroff, K.: Swiss prealpine Rietholzbach research catchment and lysimeter: 32 year time series and drought event, Water Resour. Res., 48, W06526, https://doi.org/10.1029/2011WR011749, 2012.
Shekhar, V., Stockle, D., Thellmann, M., and Vermeer, J. E. M.: The role of plant root systems in evolutionary adaptation, Plant Dev. Evol., 131, 55–80, https://doi.org/10.1016/bs.ctdb.2018.11.011, 2019.
Simard, S. W.: Mycorrhizal Networks Facilitate Tree Communication, Learning, and Memory, in: Memory and Learning in Plants, edited by: Baluska, F., Gagliano, M., and Witzany, G., Springer, 191–213, https://doi.org/10.1007/978-3-319-75596-0_10, 2018.
Singh, C., Wang-Erlandsson, L., Fetzer, I., Rockstroem, J., and van der Ent, R.: Rootzone storage capacity reveals drought coping strategies along rainforest-savanna transitions, Environ. Res. Lett., 15, 124021, https://doi.org/10.1088/1748-9326/abc377, 2020.
Smart, M. S., Filippelli, G., Gilhooly, W. P., Ozaki, K., Reinhard, C. T., Marshall, J. E. A., and Whiteside, J. H.: The expansion of land plants during the Late Devonian contributed to the marine mass extinction, Commun. Earth Environ., 4, 449, https://doi.org/10.1038/s43247-023-01087-8, 2023.
Smith, B., Wårlind, D., Arneth, A., Hickler, T., Leadley, P., Siltberg, J., and Zaehle, S.: Implications of incorporating N cycling and N limitations on primary production in an individual-based dynamic vegetation model, Biogeosciences, 11, 2027–2054, https://doi.org/10.5194/bg-11-2027-2014, 2014.
Sprenger, M., Stumpp, C., Weiler, M., Aeschbach, W., Allen, S. T., Benettin, P., Dubbert, M., Hartmann, A., Hrachowitz, M., Kirchner, J. W., McDonnell, J. J., Orlowski, N., Penna, D., Pfahl, S., Rinderer, M., Rodriguez, N., Schmidt, M., and Werner, C.: The Demographics of Water: A Review of Water Ages in the Critical Zone, Rev. Geophys., 57, 800–834, https://doi.org/10.1029/2018RG000633, 2019.
Steffen, W., Richardson, K., Rockstrom, J., Schellnhuber, H. J., Dube, O. P., Dutreuil, S., Lenton, T. M., and Lubchenco, J.: The emergence and evolution of Earth System Science, Nat. Rev. Earth Environ., 1, 54–63, https://doi.org/10.1038/s43017-019-0005-6, 2020.
Stewart-Koster, B., Bunn, S., Green, P., Ndehedehe, C., Andersen, L., Armstrong McKay, D., Bai, X., Declerck, F., Ebi, K., Gordon, C., Gupta, J., Hasan, S., Jacobson, L., Lade, S., Liverman, D., Mohamed, A., Loriani, S., Nakicenovic, N., Obura, D. and Zimm, C.: How can we live within the safe and just Earth system boundaries for blue water?, Research Square, https://doi.org/10.21203/rs.3.rs-2861426/v1, 2023.
Stocker, B. D. D., Tumber-Davila, S. J., Konings, A. G. G., Anderson, M. C. C., Hain, C., and Jackson, R. B. B.: Global patterns of water storage in the rooting zones of vegetation, Nat. Geosci., 16, 250–256, https://doi.org/10.1038/s41561-023-01125-2, 2023.
Strand, A. E., Pritchard, S. G., McCormack, M. L., Davis, M. A., and Oren, R.: Irreconcilable differences: Fine-root life spans and soil carbon persistence, Science, 319, 456–458, https://doi.org/10.1126/science.1151382, 2008.
Sugimoto, A., Yanagisawa, N., Naito, D., Fujita, N., and Maximov, T. C.: Importance of permafrost as a source of water for plants in east Siberian taiga, Ecol. Res., 17, 493–503, https://doi.org/10.1046/j.1440-1703.2002.00506.x, 2002.
Sun, G., Domec, J. C., and Amatya, D. M.: Forest Evapotranspiration: Measurement and Modelling at Multiple Scales, Forest Hydrol.: Process. Manage. Assess., 32–50, https://doi.org/10.1079/9781780646602.0032, 2016.
Sun, G., Gao, H., and Hao, L.: Comments on “Large-scale afforestation significantly increases permanent surface water in China's vegetation restoration regions” by Zeng, Y., Yang, X., Fang, N., and Shi, Z. (2020), Agr. Forest Meteorol., 296, 108213, https://doi.org/10.1016/j.agrformet.2020.108213, 2021.
Suzuki, K., Park, H., Makarieva, O., Kanamori, H., Hori, M., Matsuo, K., Matsumura, S., Nesterova, N., and Hiyama, T.: Effect of Permafrost Thawing on Discharge of the Kolyma River, Northeastern Siberia, Remote Sens., 13, 4389, https://doi.org/10.3390/rs13214389, 2021.
Taylor, B. N., Beidler, K. V., Cooper, E. R., Strand, A. E., and Pritchard, S. G.: Sampling volume in root studies: the pitfalls of under-sampling exposed using accumulation curves, Ecol. Lett., 16, 862–869, https://doi.org/10.1111/ele.12119, 2013.
Tempel, N. T., Bouaziz, L., Taormina, R., van Noppen, E., Stam, J., Sprokkereef, E., and Hrachowitz, M.: Vegetation Response to Climatic Variability: Implications for Root Zone Storage and Streamflow Predictions, EGUsphere [preprint], https://doi.org/10.5194/egusphere-2024-115, 2024.
Tromp-van Meerveld, H. J. and McDonnell, J. J.: Threshold relations in subsurface stormflow: 1. A 147-storm analysis of the Panola hillslope, Water Resour. Res., 42, W02410, https://doi.org/10.1029/2004WR003778, 2006.
Tumber-Davila, S. J., Schenk, H. J., Du, E., and Jackson, R. B.: Plant sizes and shapes above and belowground and their interactions with climate, New Phytol., 235, 1032–1056, https://doi.org/10.1111/nph.18031, 2022.
Uhlenbrook, S.: Catchment hydrology – a science in which all processes are preferential – Invited commentary, Hydrol. Process., 20, 3581–3585, https://doi.org/10.1002/hyp.6564, 2006.
van der Ent, R. J. and Savenije, H. H. G.: Length and time scales of atmospheric moisture recycling, Atmos. Chem. Phys., 11, 1853–1863, https://doi.org/10.5194/acp-11-1853-2011, 2011.
van der Ent, R. J., Savenije, H. H. G., Schaefli, B., and Steele-Dunne, S. C.: Origin and fate of atmospheric moisture over continents, Water Resour. Res., 46, W09525, https://doi.org/10.1029/2010WR009127, 2010.
van der Ent, R. J., Wang-Erlandsson, L., Keys, P. W., and Savenije, H. H. G.: Contrasting roles of interception and transpiration in the hydrological cycle – Part 2: Moisture recycling, Earth Syst. Dynam., 5, 471–489, https://doi.org/10.5194/esd-5-471-2014, 2014.
Vannoppen, W., De Baets, S., Keeble, J., Dong, Y., and Poesen, J.: How do root and soil characteristics affect the erosion-reducing potential of plant species?, Ecol. Eng., 109, 186–195, https://doi.org/10.1016/j.ecoleng.2017.08.001, 2017.
van Oorschot, F., van der Ent, R. J., Hrachowitz, M., and Alessandri, A.: Climate-controlled root zone parameters show potential to improve water flux simulations by land surface models, Eearth Syst. Dynam., 12, 725–743, https://doi.org/10.5194/esd-12-725-2021, 2021.
van Oorschot, F., van der Ent, R. J., Alessandri, A., and Hrachowitz, M.: Influence of irrigation on root zone storage capacity estimation, Hydrol. Earth Syst. Sci., 28, 2313–2328, https://doi.org/10.5194/hess-28-2313-2024, 2024.
Violle, C., Reich, P. B., Pacala, S. W., Enquist, B. J., and Kattge, J.: The emergence and promise of functional biogeography, P. Natl. Acad. Sci. USA, 111, 13690–13696, https://doi.org/10.1073/pnas.1415442111, 2014.
Wang, J., Gao, H., Liu, M., Ding, Y., Wang, Y., Zhao, F., and Xia, J.: Parameter regionalization of the FLEX-Global hydrological model, Sci. China-Earth Sci., 64, 571–588, https://doi.org/10.1007/s11430-020-9706-3, 2021.
Wang, L., Good, S. P., and Caylor, K. K.: Global synthesis of vegetation control on evapotranspiration partitioning, Geophys. Res. Lett., 41, 6753–6757, https://doi.org/10.1002/2014GL061439, 2014.
Wang, P. and Pozdniakov, S. P.: A statistical approach to estimating evapotranspiration from diurnal groundwater level fluctuations, Water Resour. Res., 50, 2276–2292, https://doi.org/10.1002/2013WR014251, 2014.
Wang, P., Niu, G.-Y., Fang, Y.-H., Wu, R.-J., Yu, J.-J., Yuan, G.-F., Pozdniakov, S. P., and Scott R. L.: Implementing dynamic root optimization in Noah-MP for simulating phreatophytic root water uptake, Water Resour. Res., 54, 1560–1575, https://doi.org/10.1002/2017WR021061, 2018.
Wang, S., Hrachowitz, M., and Schoups, G.: Multi-decadal fluctuations in root zone storage capacity through vegetation adaptation to hydro-climatic variability have minor effects on the hydrological response in the Neckar River basin, Germany, Hydrol. Earth Syst. Sci., 28, 4011–4033, https://doi.org/10.5194/hess-28-4011-2024, 2024.
Wang, T., Wang, P., Wu, Z., Yu, J., Pozdniakov, S. P., Guan, X., Wang, H., Xu, H., and Yan, D.: Modeling revealed the effect of root dynamics on the water adaptability of phreatophytes, Agr. Forest Meteorol., 320, 108959, https://doi.org/10.1016/j.agrformet.2022.108959, 2022.
Wang, X., Whalley, W. R., Miller, A. J., White, P. J., Zhang, F., and Shen, J.: Sustainable Cropping Requires Adaptation to a Heterogeneous Rhizosphere, Trends Plant Sci., 25, 1194–1202, https://doi.org/10.1016/j.tplants.2020.07.006, 2020.
Wang, Y., Jia, B., and Xie, Z.: The Effects of Dynamic Root Distribution on Land-Atmosphere Carbon and Water Fluxes in the Community Earth System Model (CESM1.2.0), Forests, 9, 172, https://doi.org/10.3390/f9040172, 2018.
Wang-Erlandsson, L., van der Ent, R. J., Gordon, L. J., and Savenije, H. H. G.: Contrasting roles of interception and transpiration in the hydrological cycle – Part 1: Temporal characteristics over land, Earth Syst. Dynam., 5, 441–469, https://doi.org/10.5194/esd-5-441-2014, 2014.
Wang-Erlandsson, L., Bastiaanssen, W. G. M., Gao, H., Jaegermeyr, J., Senay, G. B., van Dijk, A. I. J. M., Guerschman, J. P., Keys, P. W., Gordon, L. J., and Savenije, H. H. G.: Global root zone storage capacity from satellite-based evaporation, Hydrol. Earth Syst. Sci., 20, 1459–1481, https://doi.org/10.5194/hess-20-1459-2016, 2016.
Wang-Erlandsson, L., Fetzer, I., Keys, P. W., van der Ent, R. J., Savenije, H. H. G., and Gordon, L. J.: Remote land use impacts on river flows through atmospheric teleconnections, Hydrol. Earth Syst. Sci., 22, 4311–4328, https://doi.org/10.5194/hess-22-4311-2018, 2018.
Wang-Erlandsson, L., Tobian, A., van der Ent, R. J., Fetzer, I., te Wierik, S., Porkka, M., Staal, A., Jaramillo, F., Dahlmann, H., Singh, C., Greve, P., Gerten, D., Keys, P. W., Gleeson, T., Cornell, S. E., Steffen, W., Bai, X., and Rockstrom, J.: A planetary boundary for green water, Nat. Rev. Earth Environ., 3, 380–392, https://doi.org/10.1038/s43017-022-00287-8, 2022.
Wiltshire, A. J., Burke, E. J., Chadburn, S. E., Jones, C. D., Cox, P. M., Davies-Barnard, T., Friedlingstein, P., Harper, A. B., Liddicoat, S., Sitch, S., and Zaehle, S.: JULES-CN: a coupled terrestrial carbon–nitrogen scheme (JULES vn5.1), Geosci. Model Dev., 14, 2161–2186, https://doi.org/10.5194/gmd-14-2161-2021, 2021.
Xi, Q., Zhong, H., Wang, T., He, T., Gao, H., Xia, J., Wang-Erlandsson, L., Tang, Q., and Liu, J.: Spatio-temporal variation of gray-green-blue storage capacity in nine major basins of China, Chin. Sci. Bull., 66, 4437–4448, https://doi.org/10.1360/TB-2021-0381, 2021.
Xi, Q., Gao, H., Wang‐Erlandsson, L., Dong, J., Fenicia, F., Savenije, H. H. G., and Hrachowitz, M.: Terrestrial ecosystems enhance root zones in response to intensified drought, bioRxiv [preprint], https://api.semanticscholar.org/CorpusID:270205755 (last access: 11 October 2024), 2024.
Xia, J., Zhang, Y. Y., Xiong, L. H., He, S., Wang, L. F., and Yu, Z. B.: Opportunities and challenges of the Sponge City construction related to urban water issues in China, Sci. China Earth Sci., 60, 652–658, https://doi.org/10.1007/s11430-016-0111-8, 2017.
Xu, L., Chen, N., Zhang, X., Moradkhani, H., Zhang, C., and Hu, C.: In-situ and triple-collocation based evaluations of eight global root zone soil moisture products, Remote Sens. Environ., 254, 112248, https://doi.org/10.1016/j.rse.2020.112248, 2021.
Xue, Y., De Sales, F., Vasic, R., Mechoso, C. R., Arakawa, A., and Prince, S.: Global and Seasonal Assessment of Interactions between Climate and Vegetation Biophysical Processes: A GCM Study with Different Land-Vegetation Representations, J. Climate, 23, 1411–1433, https://doi.org/10.1175/2009JCLI3054.1, 2010.
Yue, W., Wang, T., Franz, T. E., and Chen, X.: Spatiotemporal patterns of water table fluctuations and evapotranspiration induced by riparian vegetation in a semiarid area, Water Resour. Res., 52, 1948–1960, https://doi.org/10.1002/2015WR017546, 2016.
Zehe, E., Loritz, R., Jackisch, C., Westhoff, M., Kleidon, A., Blume, T., Hassler, S. K., and Savenije, H. H.: Energy states of soil water – a thermodynamic perspective on soil water dynamics and storage-controlled streamflow generation in different landscapes, Hydrol. Earth Syst. Sci., 23, 971–987, https://doi.org/10.5194/hess-23-971-2019, 2019.
Zeng, X. B., Dai, Y. J., Dickinson, R. E., and Shaikh, M.: The role of root distribution for climate simulation over land, Geophys. Res. Lett., 25, 4533–4536, https://doi.org/10.1029/1998GL900216, 1998.
Zhang, F., Zou, Y.-N., and Wu, Q.-S.: Quantitative estimation of water uptake by mycorrhizal extraradical hyphae in citrus under drought stress, Sci. Hortic., 229, 132–136, https://doi.org/10.1016/j.scienta.2017.10.038, 2018.
Zhang, Y., Chiew, F. H. S., Pena-Arancibia, J., Sun, F., Li, H., and Leuning, R.: Global variation of transpiration and soil evaporation and the role of their major climate drivers, J. Geophys. Res.-Atmos., 122, 6868–6881, https://doi.org/10.1002/2017JD027025, 2017.
Zhang, Y., Liu, X., Jiao, W., Wu, X., Zeng, X., Zhao, L., Wang, L., Guo, J., Xing, X., and Hong, Y.: Spatial Heterogeneity of Vegetation Resilience Changes to Different Drought Types, Earth's Future, 11, e2022EF003108, https://doi.org/10.1029/2022EF003108, 2023.
Zhao, J., Xu, Z., and Singh, V. P.: Estimation of root zone storage capacity at the catchment scale using improved Mass Curve Technique, J. Hydrol., 540, 959–972, https://doi.org/10.1016/j.jhydrol.2016.07.013, 2016.
Zhao, R.-J.: The xinanjiang model applied in china, J. Hydrol., 135, 371–381, https://doi.org/10.1016/0022-1694(92)90096-E, 1992.
Zheng, Z. and Wang, G.: Modeling the dynamic root water uptake and its hydrological impact at the Reserva Jaru site in Amazonia, J. Geophys. Res.-Biogeo., 112, G04012, https://doi.org/10.1029/2007JG000413, 2007.
Zhou, L., Zhou, X., He, Y., Fu, Y., Du, Z., Lu, M., Sun, X., Li, C., Lu, C., Liu, R., Zhou, G., Bai, S. H., and Thakur, M. P.: Global systematic review with meta-analysis shows that warming effects on terrestrial plant biomass allocation are influenced by precipitation and mycorrhizal association, Nat. Commun., 13, 4914, https://doi.org/10.1038/s41467-022-32671-9, 2022.
- Abstract
- Introduction
- The definition of root zone
- Root zone in the Earth system
- Heterogeneities within the root zone
- Emergent behaviour of root zone as a holistic system
- Root zone in the Anthropocene
- Outlook
- Concluding remarks
- Code and data availability
- Author contributions
- Competing interests
- Disclaimer
- Acknowledgements
- Financial support
- Review statement
- References
- Abstract
- Introduction
- The definition of root zone
- Root zone in the Earth system
- Heterogeneities within the root zone
- Emergent behaviour of root zone as a holistic system
- Root zone in the Anthropocene
- Outlook
- Concluding remarks
- Code and data availability
- Author contributions
- Competing interests
- Disclaimer
- Acknowledgements
- Financial support
- Review statement
- References