the Creative Commons Attribution 4.0 License.
the Creative Commons Attribution 4.0 License.
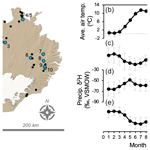
Spatiotemporal variation of modern lake, stream, and soil water isotopes in Iceland
David J. Harning
Jonathan H. Raberg
Jamie M. McFarlin
Yarrow Axford
Christopher R. Florian
Kristín B. Ólafsdóttir
Sebastian Kopf
Julio Sepúlveda
Gifford H. Miller
Áslaug Geirsdóttir
As global warming progresses, changes in high-latitude precipitation are expected to impart long-lasting impacts on Earth systems, including glacier mass balance and ecosystem structures. Reconstructing past changes in high-latitude precipitation and hydroclimate from networks of continuous lake records offers one way to improve forecasts of precipitation and precipitation–evaporation balances, but these reconstructions are currently hindered by the incomplete understanding of controls on lake and soil water isotopes. Here, we study the distribution of modern water isotopes in Icelandic lakes, streams, and surface soils collected in 2002, 2003, 2004, 2014, 2019, and 2020 to understand the geographic, geomorphic, and environmental controls on their regional and interannual variability. We find that lake water isotopes in open-basin (through-flowing) lakes reflect local precipitation, with biases toward the cold season, particularly in lakes with sub-annual residence times. Closed-basin lakes have water isotope and deuterium excess values consistent with evaporative enrichment. Interannual and seasonal variabilities of lake water isotopes at repeatedly sampled sites are consistent with instrumental records of winter snowfall; summer relative humidity; and atmospheric circulation patterns, such as the North Atlantic Oscillation. Summer surface soil water isotopes span the entire range of seasonal precipitation values in Iceland and appear to be consistently overprinted by evaporative enrichment, which can occur throughout the year, although the sampling depths were shallower than rooting depths for many plant types. This dataset provides new insight into the functionality of water isotopes in Icelandic environments and offers renewed possibilities for optimized site selection and proxy interpretation in future paleohydrological studies on this North Atlantic outpost.
- Article
(3776 KB) - Full-text XML
-
Supplement
(6136 KB) - BibTeX
- EndNote
The Arctic hydrological cycle is directly tied to broad Earth systems, such as the cryosphere and biosphere, and is expected to change rapidly with continued anthropogenic warming (Serreze et al., 2006). By the end of the century, Arctic precipitation in some regions is forecasted to increase by up to 40 % – with a progressively larger proportion of rain – due to sea ice retreat, increased local evaporation, and enhanced poleward moisture transport (Bintanja and Selten, 2014; Bintanja and Andry, 2017; Bintanja et al., 2020). As a result, long-lasting impacts on hydrology, glacier mass balances, ocean circulation, ecosystem structures, and global climate system feedbacks are expected (Douville et al., 2021). However, regional-scale predictions of future precipitation changes feature large uncertainties spatially and across models owing to large decadal-scale natural variability and inter-model differences (Bintanja et al., 2020). Developing records that quantify changes in Arctic precipitation beyond the instrumental period in Earth's history provides one means of quantifying how precipitation has changed with past climate variability and can lead to more refined predictions of future precipitation and its impact on other Earth systems (e.g., Linderholm et al., 2018; Konecky et al., 2023). Geologic records of past precipitation are, however, similarly limited at present because there are few ubiquitous proxies that clearly relate to specific aspects of hydrologic cycling (Sundqvist et al., 2014).
Lake sediments are a commonly used archive to reconstruct past environmental change due to their wide geographical distribution, sensitivity to local and regional perturbations, and continuous integration of environmental information at the catchment scale (Adrian et al., 2009). In the Arctic, various physical (e.g., grain size, sediment accumulation rates, and varves), biological (e.g., pollen, chironomids, and diatoms), and geochemical proxies (e.g., compound-specific stable isotopes) have been used to infer past patterns of precipitation from lake sediment (Linderholm et al., 2018). Of these, the hydrogen or oxygen stable-isotopic compositions of aquatic and terrestrial macrofossils and lipids are commonly used because they are abundant in lakes, and a dominant proportion of the H or O in the preserved structure is derived from plant growth water, which can then be used to estimate past water isotopes through time (Verbruggen et al., 2011; Rach et al., 2017; Holtvoeth et al., 2019). Changes in the past isotopic composition of precipitation, as recorded by water isotope proxies, can therefore yield important information on the response of regional hydrologic cycles to global changes in climate.
However, the stable-isotope signatures preserved in sedimentary archives must be carefully considered with respect to the growth habitat and reservoir of meteoric water that source organisms are likely to use, changes in the seasonal bias of precipitation, catchment geometry, and changes in landscape dynamics (Sachse et al., 2012; Cluett and Thomas, 2020; McFarlin et al., 2023). Efforts to map the spatiotemporal variability of modern lake water isotopes in highly seasonal climates have advanced our understanding of the processes that control the isotopic composition of lake water on short and long timescales (Gibson et al., 2002; Leng and Anderson, 2003; Jonsson et al., 2009; Cluett and Thomas, 2020; Gorbey et al., 2022; Kjellman et al., 2022), yet such mapping efforts have not yet been conducted in Iceland. Furthermore, while regional studies are not uncommon for lake water isotopes, similar systematic efforts are limited for soil water isotopes, meaning there is a gap in observations for the growth water utilized by terrestrial plants as it isotopically relates to precipitation. Most calibration work for compound-specific water isotope proxies (e.g., n-alkyl lipids) uses mean annual precipitation (e.g., Sachse et al., 2012), yet there are local observations that demonstrate that Arctic soil water can be seasonally biased (Cooper et al., 1991; Throckmorton et al., 2016; Bush et al., 2017; McFarlin et al., 2019; Muhic et al., 2023) and/or evaporatively enriched (Tetzlaff et al., 2018; Eensalu et al., 2023). It is also common to assume that terrestrial plant growth water is restricted to seasonal precipitation because Arctic plant growth is limited to late spring and summer (e.g., Balascio et al., 2013; Curtin et al., 2019; Kjellman et al., 2020; Thomas et al., 2020). These environmental distinctions are important because lake water (aquatic) and precipitation (terrestrial) proxies are often used comparatively to reconstruct hydrologic budgets through time, and differences in the isotopic composition of varying compounds have been used quantitatively to estimate changes in relative humidity (Rach et al., 2017) or seasonal precipitation amounts (Corcoran et al., 2021; Thomas et al, 2020).
In this study, we present modern lake, stream, and soil water δ2H and δ18O data from a network of sites across Iceland (Fig. 1a). We compare lake and soil water isotopes collected during the summer and winter field seasons in 2002, 2003, 2004, 2014, 2019, and 2020, with climatological, geomorphic, and geographic parameters to assess which factors may account for the observed isotopic variability. We also present a nearly year-long time series of lake outflow water isotopes collected in 2002. Our data show that, in general, lake water isotopes (δ2Hlake) in Iceland reflect cold-season or annual precipitation with an overprint of evaporative enrichment in closed-lake basins. The interannual variability of δ2Hlake is then related to the specific hydrologic budget (i.e., winters with differing amounts of snowfall and/or years of low relative humidity) and atmospheric circulation patterns for individual years. In contrast to lake water, surface soil water isotopes (δ2Hsoil) broadly demonstrate values that align with summer-biased precipitation and annual evaporative enrichment despite the fact that most precipitation is delivered in the autumn and winter. Collectively, these data have important implications for the interpretation of water isotope proxies in Icelandic lake sediment records and provide an objective framework within which to estimate past precipitation isotopes in local Holocene archives.
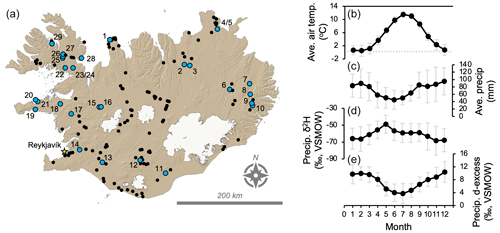
Figure 1Overview map of Iceland and modern climate. (a) Key lake and soil locations from 2014, 2019, and 2020 are shown with blue circles and are numerated (see Table 1 for site information), and additional lakes, ponds, and streams from 2002, 2003, and 2004 are shown in black (see the Supplement for site information). (b–e) Mean and standard deviation of monthly climate and water isotope data from Reykjavík, Iceland, for the years 1992–2018: (b) mean air temperature (°C) and (c) mean precipitation (mm) from the IMO (IMO, 2023) and (d) δ2H (‰, VSMOW) and (e) d-excess values (‰, VSMOW) from GNIP (IAEA/WMO, 2015).
Iceland (∼ 103 000 km2) is situated in the middle of the northern North Atlantic Ocean. Due to its location at the confluence of major oceanic and atmospheric patterns, Iceland's terrestrial climate is sensitive to variations in the strength and position of these systems (e.g., Hanna et al., 2006; Geirsdóttir et al., 2013, 2020). The southern and western coastlines of Iceland are bathed in relatively warm, saline Atlantic waters, whereas the northern and, occasionally, the eastern coastlines are influenced by cool and relatively fresh Arctic and polar waters (Stefánsson, 1962). Iceland is also proximal to the Icelandic Low, a semi-permanent center of low atmospheric pressure that forms one dipole of the North Atlantic Oscillation (NAO), along with Azores High, and is associated with frequent cyclone activity. This pressure difference between the north and south is the dominant mechanism that controls the strength and location of westerly wind and storm tracks across the northern North Atlantic (Hurrell et al., 2003). Given the different oceanographic regimes surrounding Iceland, incoming air masses are strongly influenced by the Atlantic or Arctic waters over which they originate.
The modern climate of Iceland is generally characterized by strong winds, frequent precipitation, mild winters, and cool summers (Einarsson, 1984; Hanna et al., 2004). The coldest part of Iceland is the central highlands, and snowfall in winter is more common in the northern regions. The south coast of the island is warmer, wetter, and windier compared to the northern coast (Hanna et al., 2004, 2006). In Reykjavík, southwest Iceland (Fig. 1a), temperatures average near freezing in the winter months and 10 °C in summer months (Fig. 1b, IMO, 2023). On monthly timescales, the precipitation amount in Reykjavík is inversely related to temperature, with a greater proportion of precipitation falling during autumn and winter compared to during summer (Fig. 1c, IMO, 2023). However, on longer-term decadal timescales, the precipitation amount shows a moderate positive correlation with temperature (Hanna et al., 2004). The isotopic composition of precipitation recorded at the Global Network of Isotopes in Precipitation (GNIP) station in Reykjavík is, on average, most depleted during the winter months and most enriched in the month of May, although there is large year-to-year variability (Fig. 1d, IAEA/WMO, 2015). This variability reflects the competing moisture sources to Iceland and likely explains a lack of correlation between δ2H of precipitation and air temperature (Fig. S1a in the Supplement). Deuterium excess (d-excess) values of precipitation are generally highest during the winter and lowest during the summer (Fig. 1e, IAEA/WMO, 2015). Reykjavík`s annual local meteoric water line (LMWL, Fig. 2, IAEA/WMO, 2015) of
has a similar slope but lower d-excess values than the global meteoric water line (GMWL, Craig, 1961) of
LMWLs of the different seasons (winter, DJF; spring, MAM; summer, JJA; and autumn, SON) are variable, where summer plots distinctly below the annual LMWL, while winter, spring, and autumn plot closer to and typically above the LMWL (Fig. S1b). In hydrologically closed-lake surface water systems, kinetic fractionation from evaporation will result in isotopically enriched water relative to precipitation and low d-excess values (Feng et al., 2016; Bowen et al., 2018). These are more appropriately described by a local evaporation line (LEL, Fig. 2), which plots below the LMWL (Gat, 1996).
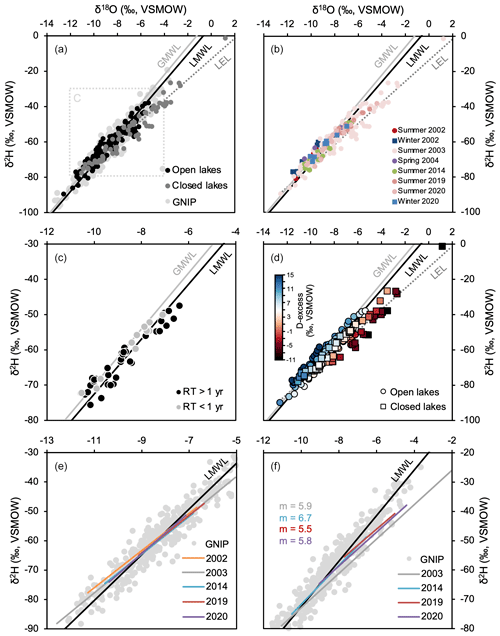
Figure 2Lake water stable isotopes from Iceland plotted against the GMWL (Craig, 1961), LMWL (GNIP Reykjavík, IAEA/WMO, 2015), and LEL (closed lakes, this study). Samples are separated by (a) hydrologic connectivity for all lakes (open and closed, n= 223); (b) year and season of collection for all lakes (n= 223); (c) residence time for open lakes from 2014, 2019, and 2020 (RT, n= 61); and (d) d-excess values for all lakes (n= 223). Panels (e) and (f) show annual LMWLs (open lakes, E) and LELs and slopes (closed lakes, f), respectively, based on lake water isotope values in comparison to the LMWL derived from GNIP data in Reykjavík (black line). Raw GNIP data are plotted as gray points.
The mountainous topography of the island, particularly in the southeast, produces a strong orographic effect on precipitation, with annual precipitation in excess of 5000 mm atop glacial summits, such as Vatnajökull and Mýrdalsjökull (Einarsson, 1984; Crochet et al., 2007). Along with southerly to southeasterly prevailing winds, precipitation amount tends to be heaviest in the south and southeast of Iceland, with a rain shadow over the central highlands, especially north of Vatnajökull (Einarsson, 1984). This geography results in distillation of air masses as they move inland and, thus, progressively more depleted δ2H values of precipitation at higher altitudes farther from the coastline (Bödvarsson, 1962; Friedman et al., 1963; Árnason, 1976). In addition, temperature and variations in moisture source also exert strong controls on precipitation isotopes in Iceland (Bödvarsson, 1962; Friedman et al., 1963) and across the North Atlantic (Dansgaard, 1964; Jouzel et al., 2000; Bowen and Revenaugh, 2003; Sodemann, et al., 2008; Steen-Larsen et al., 2015). Moisture sources in Iceland are particularly susceptible to variations of the NAO, which impacts the strength and location of westerly winds and storm tracks on interannual to decadal cycles, particularly during winter months (Hurrell et al., 2003; Olsen et al., 2012). The impact of the NAO is apparent in the modern δ2H and d-excess values of precipitation in Reykjavík, which show negative () and positive correlations (r=0.45) with the NAO index, respectively (Baldini et al., 2008).
3.1 Sample collection
We present a compilation of water isotope data collected over multiple field campaigns in 2002, 2003, 2004, 2014, 2019, and 2020 (Fig. 1a). Between 2002 and 2004, we collected lake water samples from 135 lakes and ponds (mainly in summers, with several sites being revisited in February and March; see Fig. 2b), as well as geothermal streams (n= 2), non-geothermal streams and lake water outflow (n= 8), and snow meltwater (n= 3) (Fig. S3). The sample set from 2003 (n= 148) is particularly important as it contains a large number of closed-lake basins that allow us to make robust estimates of the LEL (Fig. 2a–b). The sample sets from 2002 (n= 20) and 2004 (n= 3) are comparatively small (Fig. 2b), and since the same lakes were not revisited between the 3 years, the 2002–2004 datasets are not used to assess the detailed interannual variability of lake water isotopes. For one lake in NW Iceland (Efstadalsvatn), Ragna Aðalsteinsdóttir, the farmer residing at the site, collected water samples from the lake's outflow every 2 weeks between March and November 2002. We then visited a total of 26 lakes in summer 2014 (n= 14), summer 2019 (n= 17), winter 2020 (n= 4), and summer 2020 (n= 19) (Fig. 2b and Table 1). Of the lakes visited during the summer field season in 2014, seven were revisited in summer 2019 and 2020. All lakes visited during the summer field season in 2019 were also visited during the subsequent summer field season in 2020. This repeat-sampling strategy for 2014–2020 lake water forms the basis of our analysis focused on the interannual variability of lake water isotopes. During each field season, we sampled surface water from the center of each lake using Nalgene bottles. For select lakes in summer 2019 (n= 2) and winter 2020 (n= 4), we also sampled bottom water from the center of the lake using a Niskin water sampler. All water samples were immediately transferred to glass vials with no headspace, sealed with Teflon and/or electrical tape to prevent evaporation, and refrigerated prior to isotopic analysis. Further details on sampling locations, additional proxy data, and associated water chemistry for the 2014, 2019, and 2020 datasets can be found in Florian (2016), J. H. Raberg et al. (2021) and J. Raberg et al.(2021b), and Raberg et al. (2023).
We sampled a total of 23 surface soil samples from 18 locations in summer 2019 (Fig. 1a and Table 1). Of the 18 locations, 16 are within the catchments of lakes sampled for lake water during the same field season as described above. The two remaining soil sites (Skriðdalur and Mývatn) do not have corresponding lake water samples as they were targeted to provide forested endmembers. Soil samples were collected to evenly integrate the top 10 cm of soil. Each soil sample was sampled in 50 mL Falcon tubes, which were fully filled, sealed with Teflon tape, and kept refrigerated to prevent evaporation of water before isotopic analysis. Further details on sampling locations and additional proxy data datasets can be found in Raberg et al. (2024).
3.2 Water isotope analysis
Lake water samples were analyzed in the Stable Isotope Laboratory at the Institute of Arctic and Alpine Research, University of Colorado Boulder. Samples from 2002 to 2004 were measured via mass spectrometry methods using the CO2 equilibration method for oxygen isotopes (Epstein and Mayeda, 1953) and the uranium reduction method for hydrogen isotopes (Vaughn et al., 1998). For the remainder of the sample set, stable-isotope measurements (δ2H and δ18O) were conducted using a Picarro L2130-i cavity ring-down mass spectrometer. Each sample was run six times, with the first three data points being discarded to prevent memory isotope effects. Mean values were calculated using the final three measurements. A replicate was run after ∼20 samples to check for reproducibility. We report data using δ notation in per mil (‰) relative to the Vienna Standard Mean Ocean Water (VSMOW):
where R is 2H 1H and 18O 16O. Samples were analyzed against a suite of secondary laboratory standards that have been vigorously calibrated to primary reference materials, namely VSMOW2 and SLAP. The secondary laboratory standards (Florida water, Boulder water, and Antarctic water) have δ2H δ18O values of ‰, ‰, and ‰, and an analytical error of 1 ‰ and 0.1 ‰, respectively.
All soil samples were sent to the University of Utah Stable Isotope Research Facility for soil water vacuum line extraction and isotope measurements (see Cook et al. (2017) for methodology). Values are reported in delta notation relative to VSMOW.
Deuterium excess (d-excess) values were calculated after Dansgaard (1964) as follows:
Given that δ2H and δ18O values are generally correlative, we focus our discussion on δ2H and d-excess values for simplicity.
3.3 Additional site information
For each location, we compiled and computed additional information to test which environmental parameters are related to lake and soil water δ2H values.
For all lakes, we determined whether they were open or closed basins via the presence or absence of surface outflow in summer 2023 satellite imagery, and we measured elevation (m) and the closest distance from coast (km) using Google Earth (2023). We recognize there may be some ambiguity in this binary open–closed designation as some lakes that are, at times, open basins can be closed in late summer or in a year of low snowpack, for example. For lakes visited in 2014, 2019, and 2020 only, water depths were measured using a weighted measuring tape from the lake depocenter, and lake surface area (km2) and lake catchment area (km2) were measured in QGIS using the ÍslandsDEM (2×2 m resolution, Landmælingar Íslands). A complete model of lake bathymetries is unavailable, and so we estimate lake volumes (V, km3) for the 2014–2020 lakes based on half of an ellipsoid:
where Alake is the lake surface area, and Z is the maximum depth of the lake. Lake water residence times (RTs) were calculated after Jonsson et al. (2009):
where
with being the runoff precipitation ratio (Gibson and Edwards, 2002), where the latter is less than 1 because some precipitation that falls in a lake catchment is lost to evaporation, transpiration, sublimation, or groundwater flow. Quantitative information on these processes for Icelandic lakes is limited, and so we use an value of 0.5, which is consistent with existing Arctic lake studies conducted elsewhere (Gibson et al., 2002; Gorbey et al., 2022). We acknowledge that the value may vary spatially between sites and between years for individual lakes experiencing changes in the precipitation–evaporation balance. Additionally, the role of groundwater is an important unknown. However, as we are more broadly interested in the correlation of residence time with water stable isotopes, an estimate of 0.5 is deemed to be sufficient to address this variable. Finally, we obtained mean annual air temperature (MAT), mean annual precipitation (MAP), and seasonal means from the weather station closest to lakes sampled in 2014–2020, operated by the Icelandic Meteorological Office (IMO, 2023), including the number of years preceding sample collection equivalent to individual lake residence times. As the elevation of the closest weather station often differs from the elevation of the lake site, we also scaled temperature integrations for each lake according to the global lapse rate (6 °C km−1).
For soil sites, we used Google-Earth-derived information on the elevation and distance from the coastline, as described above for lake sites. In addition, we measured soil water content (SWC),
and in situ soil temperatures for one year using iButton loggers deployed at 10 cm soil depth in summer 2019 and collected in summer 2020 (J. Raberg et al., 2021). iButton temperatures were recorded every 3 h, which we then used to produce mean annual and seasonally integrated values. We additionally used iButtons to measure in situ surface and bottom water temperatures at 6 h intervals over the same period for four lake sites (J. Raberg at al., 2021). Finally, we include information on the dominant vegetation cover above each soil sample that we observed in the field during collection: forest (Betula pubescens); dwarf shrub (Betula nana); heath shrub (Ericaceae); moss, lichen, and/or prostrate; grass; and moss. Except for Torfavatn, which is classified as a histosol (TOC > 20 %), all collected soils are classified as brown-gleyic andosols (TOC < 12 %) (Arnalds, 2004).
3.4 Statistical analyses
We computed Pearson correlation matrices and p values to assess the relationship between water isotopes, d-excess values, and various environmental parameters using the open-source software R (R Core Team, 2022) and the corrplot package (Wei and Simko, 2021). To assess the significance of lake and soil water isotope population differences and of water isotopes and environmental variable correlations, we performed Student's t tests. We define significant results to be those with p values < 0.05.
For all lakes (2002–2020), the δ2H of lake water (δ2Hlake) ranges from −89.53 ‰ to −1.35 ‰ and generally falls within the natural range of meteoric water isotopes recorded at the Reykjavík GNIP station (Fig. 2). Spatially, δ2Hlake values tend to be more depleted in northern Iceland compared to in the south (Fig. S2). The d-excess values of lake water range from −11 ‰ to 15.71 ‰, where the values of open lakes are typically higher, ranging from −4.30 ‰ to 15.71 ‰ (Fig. 2d), and exhibit no clear spatial pattern (Fig. S2). The δ2H values of stream, snowmelt, and geothermal spring water values also fall within the natural range of meteoric water isotopes, ranging from −89.55 ‰ to −55.66 ‰, −79.11 ‰ to −60.13 ‰, and −79.37 ‰ to −73.93 ‰, respectively (Fig. S3), and the d-excess values range from −6.58 ‰ to 14.91 ‰. In general, lake water isotopes for all open lakes plot along the LMWL, whereas water isotopes for closed lakes evolve along a LEL (Fig. 2a). Using data from all closed lakes, the LEL is described by the following relationship:
Using data from 2014, 2019, and 2020, open lakes that have residence times of less than 1 year (efficient flushing) follow the annual LMWL closely. In contrast, open lakes that have residence times of over 1 year (less efficient flushing) feature larger deviations from the LMWL (Fig. 2c).
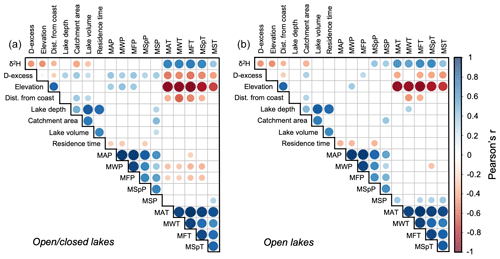
Figure 3Pearson correlation matrices for (a) all open and closed lakes and (b) only open-lake water δ2H (‰, VSMOW); d-excess values; and various climatological, geographic, and environmental parameters. Shown are elevation and distance from the coastline (n= 223); lake depth, catchment area, and lake volume (n= 80); residence time and annual and seasonal precipitation amount (n= 61); and annual and seasonal temperature (n= 80). MAP refers to mean annual precipitation, MWP refers to mean winter precipitation, MFP refers to mean autumn precipitation, MSpP refers to mean spring precipitation, MSP refers to mean summer precipitation, MAT refers to mean annual temperature, MWT refers to mean winter temperature, MFT refers to mean autumn temperature, MSpT refers to mean spring temperature, MST refers to mean summer temperature. Empty boxes reflect statistically insignificant correlations (p > 0.05). Note that lake waters sampled in 2002–2004 are only used in the regression analyses of the elevation and distance from the coast.
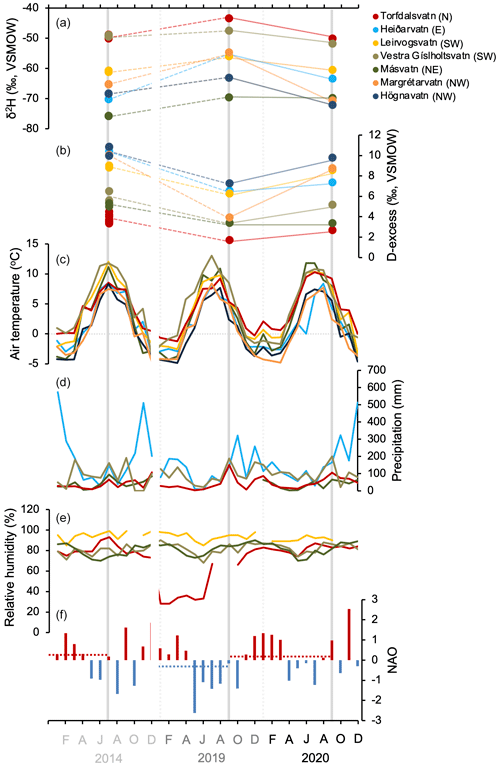
Figure 4Interannual variability of lake surface water δ2H compared with climatological datasets. (a) δ2H (‰, VSMOW) of surface water from seven lakes collected in July 2014, February 2019, and September 2020; (b) corresponding d-excess values (‰, VSMOW); (c) mean monthly air temperature (°C) for each lake; (d) mean monthly precipitation (mm) for four lakes (not available for Leirvogsvatn, Margrétarvatn, and Högnavatn); (e) mean monthly relative humidity (%) for four lakes (not available for Heiðarvatn, Margrétarvatn, and Högnavatn); and (f) mean monthly NAO (NOAA National Centers for Environmental Information, 2023). For (f), NAO annual means are denoted by dashed horizontal lines and are calculated based on the 12 months prior to lake water sampling (red indicates positive, and blue indicates negative).
For all 2014–2020 lakes (open and closed), we find strong correlations between δ2Hlake and mean annual (r= 0.66, p < 0.05), mean winter (r= 0.67, p < 0.05), mean autumn (r= 0.69, p < 0.05), and mean spring temperatures (r= 0.64, p < 0.05) and a weak but still significant correlation with mean summer temperature (r= 0.38, p < 0.05) (Fig. 3a). However, we find no significant correlations between mean annual precipitation amount and δ2Hlake values, nor do we find any correlation with seasonal precipitation amount (Fig. 3a), which may be in part due to the inherent spatial variability of precipitation and the use of weather stations with varying distances to lakes. If closed-lake basins are excluded, we find similar relationships between δ2Hlake and temperature (Fig. 3b). However, excluding closed lakes also reveals that open lakes are weakly correlated with mean spring (r= 0.37, p < 0.05) and mean summer precipitation amounts (r= 0.33, p < 0.05) (Fig. 3b). Using the entire 2002–2020 lake dataset, we find a moderate correlation between δ2Hlake values and elevation (r= -0.46, p < 0.05) and a weak correlation between δ2Hlake values and the distance from the coastline (, p < 0.05) (Fig. 3). Lake water d-excess values show weak to moderate correlations with most variables in all lakes, including elevation, the distance from the coast, precipitation amount, and temperature (Fig. 3a). However, when closed lakes are excluded, d-excess values are no longer correlated with precipitation amounts, except for mean autumn precipitation (, p < 0.05) (Fig. 3b). For repeatedly sampled lakes, summer δ2Hlake values are relatively more enriched in 2019 than summer δ2Hlake values in 2014 and 2020 (Figs. 4a and 5). Summer d-excess values are also relatively lower in 2019 compared to in 2014 and 2020 (Figs. 4b and 5). For the four lakes that have seasonal data from 2019 and 2020, winter surface δ2Hlake values are more depleted than summer surface δ2Hlake values, with differences ranging from −0.54 % to 16.51 % (Fig. 6a). Winter lake water d-excess values are relatively higher than summer d-excess values, except for one lake, Stóra Viðarvatn (Fig. 6b). For the 2002 lake outflow stream time series from Efstadalsvatn (Fig. 1a), δ2H values range from −78.75 ‰ at the end of April to −70.68 ‰ at the end of June and are generally 2H-enriched between June and August (Fig. 7a), and d-excess values range from 11.13 ‰ to 14.29 ‰, with the highest values being obtained in June (Fig. 7b).
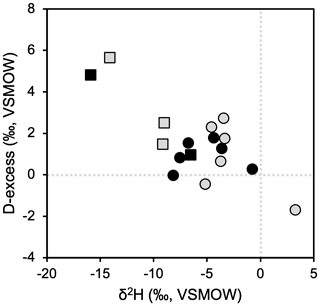
Figure 5Difference in September 2020 and 2019 lake surface water δ2H (‰, VSMOW) vs. d-excess values (‰, VSMOW) from 17 lakes. Squares denote lakes located on the NW highlands (Fig. 1), and black and gray fillings denote lakes with residence times > 1 and < 1 year, respectively.
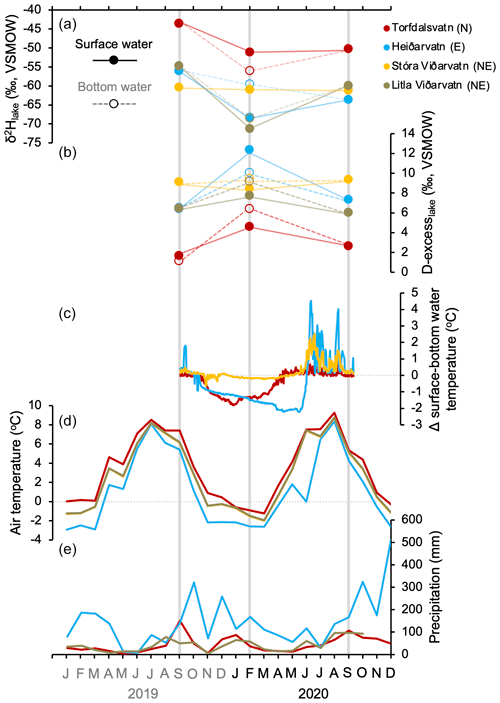
Figure 6Interannual variability of lake surface and bottom water δ2H compared with climatological datasets. (a) δ2H (‰, VSMOW) of surface and bottom water from four lakes collected in September 2019, February 2020, and September 2020; (b) corresponding d-excess values (‰, VSMOW); (c) difference between in situ lake surface and bottom water temperature (°C) (not available for Litla Viðarvatn; J. Raberg et al., 2021); (d) mean monthly air temperature (°C) for each lake; and (e) mean monthly precipitation (mm) for each lake (note that Litla Viðarvatn is the same as Stóra Viðarvatn).
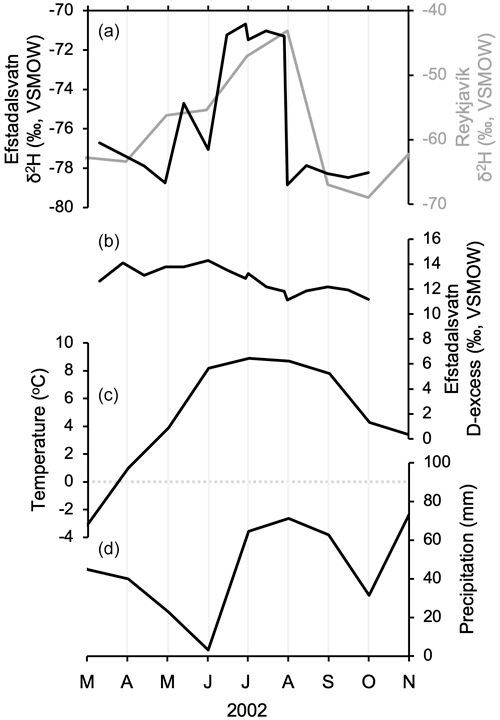
Figure 7Time series of (a) stream outflow δ2H (‰, VSMOW) and (b) d-excess values (‰, VSMOW) from Efstadalsvatn between March and October 2002 in comparison to local instrumental weather data: (c) air temperature (°C) and (d) precipitation (mm). Reykjavík GNIP precipitation δ2H (‰, VSMOW) is shown in panel (a) (gray) for comparison (IAEA/WMO, 2015).
The δ2H values of surface soil water (δ2Hsoil) from 2019 range from −91.34 ‰ to −31.71 ‰ and fall within the natural range of meteoric water isotopes recorded at the Reykjavík GNIP station across all seasons (Fig. 8). Due to the small size of the δ2Hsoil dataset compared to lakes, it is difficult to assess if there is any clear spatial variability (Fig. S4). Soil water d-excess values range from −5.04 ‰ to 9.84 ‰ (Fig. 8b). In general, surface soil water isotopes fall below the LMWL, although samples collected from beneath densely vegetated moss or grass cover plot along the LMWL (Fig. 8). Five of the sites have two soil samples from the same lake catchment, with the difference in δ2Hsoil values ranging from 1.11 ‰ to 21.65 ‰. In general, surface soil waters are 2H-enriched compared to surface lake waters from the same year, although four soil water samples are 2H-depleted compared to surface lake water at the same locations. For temperature, we find a moderate correlation between δ2Hsoil and in situ soil mean summer temperature (r= 0.51, p < 0.05) and no significant correlations between mean annual, mean winter, mean autumn, and mean spring temperature and δ2Hsoil values (Fig. 9). For precipitation, we find a moderate correlation between δ2Hsoil and mean summer precipitation amount (r= 0.56, p < 0.05) and no significant correlation between mean annual, mean winter, mean autumn, and mean spring precipitation amount and δ2Hsoil values (Fig. 9). In contrast, soil water d-excess values are positively correlated with mean annual (r= 0.58, p < 0.05), mean winter (r= 0.61, p < 0.05), mean autumn (r= 0.56, p < 0.05), and mean spring precipitation amounts (r= 0.50, p < 0.05) but not with the mean summer precipitation amount (Fig. 9). We also find weak to moderate correlations between d-excess values and mean annual (r= 0.58, p < 0.05), mean autumn (r= 0.57, p < 0.05), mean spring (r= 0.50, p < 0.05), and mean summer precipitation amounts (r= 0.33, p < 0.05) (Fig. 9). We find moderate correlations between δ2Hsoil and elevation (, p < 0.05) and between d-excess values and elevation (r= 0.43, p < 0.05) and no significant correlation with the distance from the coast (Fig. 9). Finally, we find no significant correlation between δ2Hsoil and soil water content (Fig. 9).
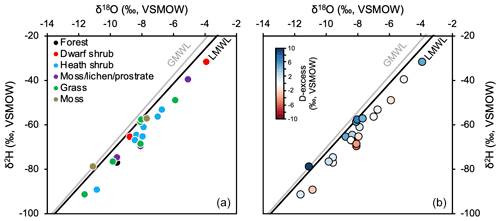
Figure 8Soil water stable isotopes (n= 23) plotted against the GMWL and LMWL. (a) Samples colored according to the overlying vegetation as described during collection in September 2019 and (b) samples colored by d-excess values (‰, VSMOW).
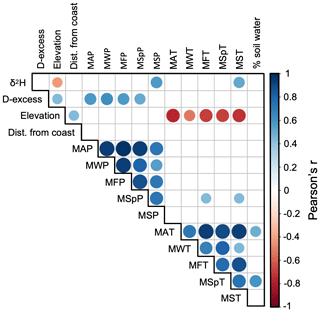
Figure 9Pearson correlation matrix of 2019 soil water δ2H (‰, VSMOW); d-excess values; and various climatological, geographic, and environmental parameters (n= 23). Empty boxes reflect statistically insignificant correlations (p > 0.05).
Student's t tests for lake and soil water populations show that δ2H and δ18O values are not statistically different between the two groups (p > 0.05). However, Student's t tests for d-excess values, which take in account both δ2H and δ18O variables, show that lake and soil water populations are statistically different from one another (p < 0.05). This supports the separate assessment of lake and surface soil water isotopes in Iceland.
5.1 Lake water isotopes
5.1.1 Environmental controls
Icelandic lake water isotopes from 2002 to 2020 generally plot close to or on the LMWL (estimated using GNIP data from Reykjavík, IAEA/WMO, 2015), demonstrating that most lake water in Iceland is isotopically consistent with unmodified precipitation and a low-temperature, high-precipitation environment. Lakes with δ2Hlake values that generally plot on the LMWL are all open lakes (n= 149, Fig. 2a) with active inflows providing recharge from meteoric water that falls in the catchment and is stored according to lake residence times (Jonsson et al., 2009). For 2014 to 2020 lakes, the strongest predictor of δ2Hlake in open lakes is cold-season temperatures: winter (r= 0.53, p < 0.05), autumn (r= 0.62, p < 0.05), and spring (r= 0.63, p < 0.05) (Fig. 3b). However, due to the lack of correlation between GNIP δ2H values of precipitation and air temperature (Fig. S1a), we do not interpret δ2Hlake as a function of temperature but rather a function of precipitation sourced from cool seasons. This is supported by the fact that open lakes with residence times of less than 1 year (efficient flushing) plot close to the LMWL (Fig. 2c), which was found to be biased towards the cold season in Reykjavík GNIP precipitation data (Fig. S1b), and the fact that a larger proportion of precipitation is delivered in autumn and winter (Fig. 1c). Surprisingly, we observe no relationship between precipitation amount and δ2Hlake (Fig. 3b), which may be due to the large standard deviation of seasonal precipitation amounts recorded at the Reykjavík GNIP station (Fig. 1c). Open lakes that have residence times of greater than 1 year (less efficient flushing) deviate from the LMWL more than lakes with residence times of less than 1 year (Figs. 2c and S1). Following the seasonal bias of LMWLs (Fig. S1b), this indicates that the dominant proportion of precipitation falling in these lake catchments falls in the cold season (plots close to annual LMWL) and possibly in summer (plots below annual LMWL). For the latter “summer” lakes, the low corresponding d-excess values imply that there is some degree of seasonal evaporation (Fig. 2d), meaning that precipitation may still be dominantly sourced from cold seasons but that repeated episodes of evaporation may obscure the seasonal signal in lakes with longer residence times.
Several other mechanisms also help to explain the trends observed in Icelandic lake water isotopes. First, 49 lakes in the entire dataset (2002 to 2020) are closed basins, meaning that the meteoric water that falls in the catchment is contained until it is lost through evaporation. In Iceland, the effect of evaporation (kinetic fractionation) is observed through the deviation of closed lakes' water isotope values along the LEL and relatively low d-excess values (Fig. 2d). Given the maritime and humid climate of Iceland, as well as the fact that some of the evaporative lakes are located on the coast, this observation contrasts with documented lake water isotopes in the higher-latitude Arctic, where evaporation is minimal in coastal lakes (Leng and Anderson, 2003; Cluett and Thomas, 2020; Kjellman et al., 2022). Compared to these other Arctic lake studies, we hypothesize that the relatively warmer temperature, low amounts of summer precipitation, and high wind speeds in Iceland, particularly along the coast and in the highlands (Einarsson, 1984), may contribute to enhanced seasonal evaporation during the relatively longer open-water season in these lakes (e.g., Feng et al., 2016; Gonfiantini et al., 2020). In addition, as winds are typically strongest in winter (Einarsson, 1984), a partially ice-free cool season, when the water–air temperature difference is greatest, may contribute to enhanced lake water evaporation as well. Second, we observe moderate and weak correlations in all lakes (2002 to 2020) between δ2Hlake and two geographic parameters, namely elevation (r= −0.46, p < 0.05) and the distance from the coastline (r= −0.33, p < 0.05) (Fig. 3a). Icelandic lakes that are further inland tend to be at higher elevations; thus, Rayleigh distillation is also a control on the spatial variability of lake water isotopes in Iceland, consistently with other surface water, near-surface groundwater, and precipitation stable-isotope studies (Bödvarsson, 1962; Friedman et al., 1963; Árnason, 1976; Stefánsson et al., 2019).
5.1.2 Interannual variability
LMWLs calculated from open lakes in the years 2002, 2003, 2014, 2019, and 2020 reveal similarities and differences that are likely to be driven by broad annual changes in climate across Iceland (Fig. 2e). First, for open lakes, we observe that 2002 stands out with a LMWL slightly above the other years, which are all similar in slope and intercept (Fig. 2e). The samples from 2002 were all collected from the northwestern peninsula, which is characterized by a cooler climate and a greater proportion of precipitation falling as snow (Einarsson, 1984). Following our seasonal LMWL analysis of Reykjavík GNIP data, the higher intercept of the 2002 lake water LMWL relative to the GNIP LMWL is consistent with a greater proportion of isotopically depleted winter precipitation (Fig. S1b). The lower intercepts of lake water LMWLs across the other years (2003, 2014, 2019, and 2020; Fig. 2e) are consistent with spring and autumn LMWLs (Fig. S1b) and likely reflect the inclusion of lakes that receive relatively lower amounts of winter snow. In any case, these data demonstrate that, broadly, the seasonality of lake water isotopes is consistent with cold-season precipitation.
LELs calculated from closed lakes in the years 2003, 2014, 2019, and 2020 also reveal similarities and differences that are likely to be driven by broad changes in Icelandic climate (Fig. 2f). The intersection of the LEL, defined based on closed-lake basin water isotopes, and the LMWL of precipitation is commonly used to infer the isotopic composition of lake water input prior to evaporative enrichment (e.g., Gibson et al., 2016). The annual LELs show that 2019 has the highest LEL–LMWL intercept, followed by 2020, 2014, and 2020, all of which fall within the range of GNIP data from Reykjavík (Fig. 2f). Some high-latitude studies infer such changes to reflect changes in the seasonality of precipitation, where higher (lower) LEL–LMWL intercepts reflect more summer (winter) precipitation (e.g., Kjellman et al., 2022). However, the seasonality of Reykjavík precipitation spans the range of LEL–LMWL intercepts and, as previously noted, is better reflected in seasonal LMWLs (Fig. S1b). However, we do note that the slope of the annual LELs derived from lake water isotopes varies between years, where 2019 is the shallowest (m= 5.5), followed by 2020 (m= 5.8), 2003 (m= 5.9), and 2014 (m= 6.7) (Fig. 2f). Under the assumption that LELs are predominately the result of variations in the precipitation–evaporation balance and that all lakes respond similarly, the slope of LELs can be used as an indicator of relative humidity variability in high-latitude lakes, where lower slopes reflect increased lake water evaporation (e.g., Leng and Anderson, 2003; Kopec et al., 2018). Hence, these data suggest that 2019 was relatively dry and that 2014 relatively wet compared to 2003 and 2020.
Seven lakes in Iceland were sampled for surface lake water isotopes during September 2014, 2019, and 2020 and demonstrate interannual δ2Hlake variability ranging from 0.24 (Margrétarvatn, NW Iceland) to 17.88 ‰ (Heiðarvatn, E Iceland) (Fig. 4a) and d-excess variability ranging from 1.69 (Torfdalsvatn, N Iceland) to 10.83 (Högnavatn, NW Iceland). In all seven lakes, δ2Hlake values in 2019 were relatively enriched and had lower d-excess values than in 2014 and 2020. For some lakes, winter snowfall may be a factor explaining δ2Hlake values, particularly for those at higher elevations. At Heiðarvatn (442 m a.s.l., E Iceland), 2H-depleted snow from the preceding winter may have yielded a winter-biased signal that lingered into the summer season for some years. We note that the winter preceding September 2014 featured substantially higher amounts of winter snowfall (1063 mm) than the winter preceding September 2019 (553 mm) at Heiðarvatn (Fig. 4d), which is consistent with the relatively 2H-depleted values in 2014 (17.88 ‰, Fig. 4a). For the low-elevation, coastal lake Torfdalsvatn (N Iceland), the amount of winter precipitation did not vary substantially between 2014 and 2020, as it did for Heiðarvatn. However, local relative humidity at Torfdalsvatn was significantly lower through the winter, spring, and summer in 2019 compared to in 2014 and 2020 (Fig. 4e). As Torfdalsvatn is a closed lake, the relative enrichment of δ2Hlake values and the lower d-excess values in 2019 (Fig. 4a–b) are consistent with the relatively dryer conditions experienced during the same year.
A total of 17 lakes were sampled in September of both 2019 and 2020 and revealed interannual variability, with δ2Hlake value differences between 2019 and 2020 ranging from −3.31 (Skjaldarvatn, W Iceland) to 15.89 ‰ (Margrétarvatn, NW Iceland) and d-excess value differences ranging from -1.70 (Skjaldarvatn, W Iceland) to 5.65 ‰ (Berufjarðarvatn, NW Iceland) (Fig. 5). In general, we observe lower δ2Hlake values and higher d-excess values in 2020 compared to in 2019, except for one lake (Skjaldarvatn), which has higher δ2Hlake values and lower d-excess values in 2020 (Fig. 5). We predicted that lakes with residence times of less than 1 year (efficient flushing) would feature larger interannual variability (e.g., Gibson et al., 2002; Leng and Anderson, 2003). However, lake residence time does not correlate with the magnitude of the δ2Hlake value difference between 2019 and 2020 (Fig. 5). However, we do observe greater δ2Hlake and d-excess difference for lakes in the NW highlands (squares) compared to for lakes elsewhere in Iceland (circles, Fig. 5). We note that, during the 2020 sample collection, there was fresh snow accumulation in the catchments of the NW highland lakes in September, whereas we did not observe this for the same time period in 2019 (Fig. S5). This is consistent with more 2H-depleted values in 2020 relative to 2019; thus, snowmelt at high elevations may partially explain the relatively 2H-depleted values for NW highland lakes compared to lower-elevation sites elsewhere in Iceland.
Finally, given that lake water isotope values generally reflect unmodified precipitation (Fig. 2), the precipitation source also likely impacts the Icelandic δ2Hlake values. Variation of the NAO will impact the strength and location of westerly winds and storm tracks over interannual to decadal cycles. In NAO+ (strong low pressure over Iceland), the westerlies center over Iceland, resulting in warm and moist air being delivered from the south. Conversely, during NAO− (weak low pressure over Iceland), the storm track shifts southward, resulting in relatively cooler and drier conditions in Iceland (Hurrell et al., 2003). Therefore, we would expect that, during NAO+ (NAO−) years, lake water in Iceland may be 2H-enriched (2H-depleted) in through-flowing lakes due to different source regions from the south and north, respectively, which can differ isotopically by 100 ‰ (Bowen and Revenaugh, 2003; Hurrell et al., 2003). In closed-lake basins, we may expect 2H enrichment and lower d-excess values of lake water in NAO− years due to the drier conditions that promote evaporation. In 2019, the average NAO index for the 12 months preceding lake water sample collection was negative (−0.21), whereas those for 2014 and 2020 were positive (0.23 and 0.09, respectively; Fig. 4f, NOAA National Centers for Environmental Information, 2023). Our dataset demonstrates more 2H-enriched water isotopes with lower d-excess values in 2019 relative to 2014 and 2020 (Fig. 4a–b). For through-flowing lakes, the 2H enrichment in 2019 may be the result of less 2H-depleted winter snowfall in a NAO− year, whereas, for closed lakes (e.g., Torfdalsvatn), the 2H enrichment and low d-excess values may additionally reflect evaporative enrichment resulting from the changes in relative humidity induced by NAO− conditions. For the latter, this is also consistent with the relatively lower LEL slope in 2019 (Fig. 2f), which has previously been shown to correlate with NAO variability in lakes in western Greenland (Kopec et al., 2018). In any case, annual changes in the NAO index and the associated changes in moisture source and relative humidity are consistent with the isotopic variability of lake water in Iceland, demonstrating that the precipitation source is likely a contributing influence in relation to δ2Hlake values across Iceland on at least interannual timescales.
5.1.3 Seasonal variability
Four lakes were sampled for surface lake water three times in two different seasons between 2019 and 2020: September 2019, February 2020, and September 2020. In addition, we took bottom water samples from two lakes in September 2019 (Torfdalsvatn and Heiðarvatn) and from all four lakes in February 2020 (Torfdalsvatn, 4.4 m depth; Heiðarvatn, 13 m depth; Stóra Viðarvatn, 19.8 m depth; and Litla Viðarvatn, 1.5 m depth) (Fig. 6a). In general, we observe more depleted surface δ2Hlake values in winter, which likely reflects the seasonal change in precipitation, where winter snow is isotopically depleted relative to rain, which dominates the other seasons (Fig. 6a). In addition, winter d-excess values of surface water are higher than in summer, except in Stóra Viðarvatn, which may reflect varying degrees of summer evaporation in closed (Torfdalsvatn) and even open lakes (Heiðarvatn and Litla Viðarvatn). Compared to surface lake water, winter bottom δ2Hlake values are relatively depleted in Torfdalsvatn, relatively enriched in Heiðarvatn and Litla Viðarvatn, and equivalent in Stóra Viðarvatn (Fig. 6a). Winter bottom water d-excess values are relative higher in Torfdalsvatn and Litla Viðarvatn, lower in Heiðarvatn, and similar in Stóra Viðarvatn (Fig. 6b). While the difference varies between lakes, the general trends are likely to reflect the winter stratification of the water columns. Using in situ daily lake water temperatures from the same depths that δ2Hlake and d-excess values are from for three of the lakes (Torfdalsvatn, Heiðarvatn, and Stóra Viðarvatn; Fig. 6c, J. Raberg et al., 2021), we observe strong winter water column stratification in Torfdalsvatn and Heiðarvatn but not in Stóra Viðarvatn, which is consistent with differences in surface and bottom δ2Hlake and d-excess values, where Torfdalsvatn and Heiðarvatn's δ2Hlake values differ by 4.92 ‰ and 8.62 ‰, respectively, while Stóra Viðarvatn's values differ only by 0.06 ‰ (Fig. 6a). For Litla Viðarvatn, in the absence of continuous bottom water temperature measurements, sonde water quality measurements (i.e., lake water temperature, pH, dissolved oxygen, and conductivity) from September 2019 and February 2020 (Raberg et al., 2023) demonstrate that this lake is also stratified during the winter (Fig. S6), which is consistent with the difference between surface and bottom water δ2Hlake values of 2.74 ‰ (Fig. 6a).
In addition to seasonal δ2Hlake values, we also collected bimonthly water samples from the outflow of a low-elevation lake in northwestern Iceland (Efstadalsvatn, Fig. 7a, black) between March and November 2002. Efstadalsvatn has a short residence time of ∼0.1 per year (Table 1), meaning that meteoric water should be efficiently recharged through the lake. Compared to the seasonal changes in precipitation δ2H values in Reykjavík for the same year (Fig. 1d), the δ2H values of the outflow water are relatively dampened, ranging from −78.75 ‰ at the end of April to −70.68 ‰ at the end of June (Fig. 7a, black), but these are similar in scale compared to the interannual and seasonal variability observed in δ2Hlake values from other lakes (Figs. 4–6). Notably, we observe relatively 2H-depleted values between March and April, followed by rapid (14 d) 2H enrichment of 5.83 ‰ at the beginning of June and then rapid (2 d) 2H depletion of 7.56 ‰ at the end of August, after which δ2H values are stable and low (Fig. 7a, black). The relatively 2H-depleted values in March and April may be due to the seasonal variability of precipitation δ2H values in Reykjavík (Fig. 7a, gray) and/or lingering snowmelt in the catchment associated with relatively cool seasonal air temperatures (Fig. 7c). The 2H enrichment observed at the beginning of June occurs at the same time as an increase in the local precipitation amount (Fig. 7d), which is likely to be in the form of rain (as opposed to 2H-depleted snow) due to above-freezing air temperatures (Fig. 7c). Evaporative enrichment is not likely to explain this step change as Efstadalsvatn has an open catchment and a short residence time, and d-excess values are high and inconsistent with a kinetic fractionation effect (Fig. 7b). This 2H enrichment of lake outflow water is also superimposed over a more gradual 2H enrichment of Reykjavík precipitation values (Fig. 7a). The cause of the rapid 2H depletion at the end of August may be related to the source and/or seasonal variability of precipitation δ2H values. In Reykjavík, monthly averages show a large depletion between August and September (Fig. 7a, gray). As precipitation sources may differ between Reykjavík (southwest) and Efstadalsvatn (northwest), these similarities are difficult to confidently correlate. In any case, while there are no data for Efstadalsvatn, surface and bottom water isotopes and temperatures from Torfdalsvatn, a similarly shallow lake (3.8 m vs. 5.8 m depth, respectively; Caseldine et al., 2003), show that it is dimictic and seasonally stratified from early April through October (Fig. 6a–c). This suggests that Efstadalsvatn could also be seasonally stratified, resulting in August rainfall flushing through the lake surface without mixing with the deeper water (e.g., Boehrer and Schultze, 2008).
5.2 Surface soil water isotopes
Isotopes of surface soil water collected in summer in Iceland span the entire range of seasonal precipitation values for Iceland, suggesting that the seasonality of source water and/or the residence time of soil water are spatially variable across the island. Values correlate significantly with mean summer environmental parameters, i.e., precipitation amount (r= 0.56, p < 0.05) and temperature (r= 0.52, p < 0.05) (Fig. 9), suggesting that summer is the source water season for at least some sites and that there is, broadly, more integration of warm-season precipitation than of lake water across the soil water dataset. In contrast, d-excess values correlate with mean annual (r= 0.58, p < 0.05), mean winter (r= 0.61, p < 0.05), mean autumn (r= 0.56, p < 0.05), and mean spring precipitation amounts (r= 0.50, p < 0.05) (Fig. 9), demonstrating that the input of seasonal precipitation to soils in Iceland is not clear-cut. As most Icelandic surface soil water isotopes plot below the LMWL and have low to negative d-excess values (Fig. 8), the pattern of soil waters isotopes, regardless of source water seasonality, is broadly consistent with modification via evaporation. While the residence time of Icelandic soil water is unknown, the residence time of soil water in other wet, low-energy North Atlantic locations is typically controlled by soil type and overlying vegetation (Tetzlaff et al., 2014; Geris et al., 2015a, b; Sprenger et al., 2017). The andic properties of the soil types in Iceland (i.e., histosol and brown-gleyic Andosol) result in relatively high water retention (Arnalds, 2004), which is consistent with the correlations of precipitation and d-excess values throughout the year. As soil temperatures at these sites periodically dip below freezing during winter months (Fig. S7), seasonal freezing of the ground may contribute to the integration of summer precipitation δ2Hsoil values, despite lower summer precipitation amounts, by preventing the incorporation of cold-season water into a frozen-water–soil matrix at some sites. Alternatively, surface soil water residence times at some sites could just be short enough that, by summer sampling, winter precipitation has percolated deeper into the soil profile. When soil samples are separated by the type of vegetation cover, we observe that moss- and grass-covered soil samples associated with marsh environments plot closer to the LMWL and have higher d-excess values compared to other types of vegetation cover (Fig. 8). This likely reflects the saturated marsh environment that these soils were sampled from, which have high water tables, retain water for longer than soils with efficient drainage, and are less prone to evaporation.
For five locations, we took two surface soil samples from the same lake catchments but different locations, as measured for lake water isotopes (Heiðarvatn, Másvatn, Stóra Viðarvatn, Systravatn, and Torfdalsvatn). The δ2Hsoil value differences for the samples from the same lake catchments range from 1.11 (Systravatn) to 21.65 ‰ (Heiðarvatn), and corresponding d-excess value differences range from 0.54 (Systravatn) to 5.66 (Heiðarvatn). The variability observed in δ2Hsoil and d-excess values from the same location suggests that additional processes beyond climate and geography contribute to soil water isotopic variability in Iceland. This heterogeneity may be one reason why correlations are weaker between δ2Hsoil values and mean summer temperature (r= 0.52, p < 0.05), precipitation amount (r= 0.52, p < 0.05), and elevation () (Fig. 9) compared to the same correlations found with δ2Hlake values (Fig. 3). While vegetation cover may explain some spatial variability in the broader Icelandic dataset, it likely does not account for intersite variability. For example, the sites with high intersample δ2Hsoil value differences (Heiðarvatn, with 21.65 ‰, and Stóra Viðarvatn, with, 11.37 ‰) are both covered by the same vegetation type (moss and heath shrub, respectively), while the only location that had different vegetation cover (Másvatn, grass and heath shrub) had one of the lowest δ2Hsoil value differences (2.04 ‰). Variations in snow cover duration may be another possible explanation for inter-site variability. However, soil temperatures (3 h resolution) for the following year show that sites with larger δ2Hsoil value differences (e.g., Heiðarvatn and Stóra Viðarvatn) have melt-out differences of 3 and 8 d, respectively, whereas sites with small δ2Hsoil value differences (e.g., Másvatn, Systravatn, and Torfdalsvatn) show melt-out differences with a larger range from 3 to 13 d (Fig. S7). Therefore, the parameters for which we have data, i.e., local climate parameters, fine-scale observations of vegetation cover, and in situ thermal regimes, do not adequately explain the site-to-site variability of surface soil water isotopes.
5.3 Implications for paleoclimate proxies
Lake water isotope proxies have become an increasingly common tool to infer past changes in the high-latitude hydrologic cycle, including the seasonality of precipitation (e.g., Kjellman et al., 2020; Thomas et al., 2020; Corcoran et al., 2021), moisture balance (e.g., Anderson and Leng, 2004; Balascio et al., 2018), and precipitation source (e.g., Cowling et al., 2022; van der Bilt et al., 2022). Foundational to any paleohydrological study is a clear understanding of the local environmental controls behind water isotope variability. For Icelandic lake water, we find that cold-season precipitation, lingering snowpack in fringe seasons, summer relative humidity, and NAO cyclicity are important modulators of δ2Hlake on interannual timescales, particularly for lakes with sub-annual residence times. These are important considerations as they indicate that the seasonality of runoff may not always align with the seasonality of precipitation. Combined with the differences we observe between surface and bottom water δ2Hlake, the complexities of δ2Hlake are consistent with the poorer relationships observed between typically aquatic mid-chain plant waxes and δ2Hlake values vs. typically terrestrial long-chain plant waxes and δ2H of precipitation values (McFarlin et al., 2019). Surface soil water isotopes are highly variable across the island, with only some sites being supportive of previous assumptions that terrestrial biomarker water isotopes reflect summer precipitation (e.g., Balascio et al., 2013; Curtin et al., 2019; Kjellman et al., 2020; Thomas et al., 2020). However, we stress that these soil water isotopes are from the surface (i.e., upper 10 cm of the soil), and evidence suggests that high-latitude plant roots may source water from the surface (Amin et al., 2020), as well as from below the surface layer (e.g., Eensalu et al., 2023). Therefore, these data may or may not reflect broader plant growth water, and the enrichment observed in Icelandic surface soils also may not reflect water found in deeper soils. Hence, while still valuable, the soil data currently provide a limited perspective on the soil water isotope systematics needed to inform plant growth and terrestrial leaf wax water isotope studies in Iceland.
In Iceland, there are only two Holocene records of paleo-precipitation developed from water isotopes proxies, one using aquatic invertebrates (i.e., chironomids) from a lake in northeastern Iceland (Stóra Viðarvatn, Wooller et al., 2008) and another using leaf wax n-alkanes from a fjord in northwestern Iceland (Moossen et al., 2015). Both records observe inconsistencies with known temperature variability and, through multi-proxy comparisons, infer that the changes in water isotopes were related to precipitation. The dominant controls that we find in modern lake water isotopes support prior assumptions that chironomid δ18O values from Stóra Viðarvatn encode information about past changes in precipitation seasonality and moisture source (Wooller et al., 2008). However, we do note that Stóra Viðarvatn has one of the longest residence times in the dataset (∼17 years, Table 1), and the lake's modern water isotopes appear to be insensitive to interannual climate variability (Fig. 6). While residence time is not a strong predictor of lake water isotope variability in Iceland, the relative insensitivity of Stóra Viðarvatn to annual changes in moisture supply likely results in a decadally averaged paleohydrological record. On the other hand, the fjord record from northwestern Iceland is based on terrestrial leaf waxes that were subsequently transported to the fjord (Moossen et al., 2015), which are complicated by unknown transport and residence times, as well as by uncertainties in marine age model reservoir corrections. The relatively limited perspective that our modern surface soil water isotopes provide regarding terrestrial biomarker water isotope proxies also makes it challenging to interpret the fjord record, although it remains likely that the fjord leaf wax δ2H values are summer biased. Looking forward, the strong linear relationship between lake water and aquatic-invertebrate (e.g., chironomids) oxygen isotopes (Verbruggen et al., 2011) suggests that their application in Icelandic lake sediment records is promising. However, further calibration of terrestrial and aquatic plant waxes, their isotopic variability, their relationship to source waters, and their relative contribution to the sedimentary pool is vital to further advance the use of biomarker-derived water isotope proxies in Icelandic sedimentary records (e.g., McFarlin et al., 2019; Dion-Kirschner et al., 2020; Hollister et al., 2022).
Our modern lake and soil water isotope dataset provides important insights into the impact that various geographic, geomorphic, and environmental variables have on the distribution of water isotopes across Iceland. Importantly, the data open up the possibility for optimized site selection and more informed paleohydrological studies in Iceland. For example, we identify lakes that are sensitive to evaporative enrichment, whose sediments can be useful in reconstructing Holocene moisture balances, and lakes that record unmodified meteoric water, which offers the possibility of reconstructing changes in precipitation seasonality and moisture source related to atmospheric patterns. Collectively, these paleohydrological records can help address open questions related to, for example, the impact of precipitation on the Holocene mass balance of Icelandic ice caps and shifts in terrestrial plant communities. As both glaciers and terrestrial plants are part of positive feedback loops in the climate system through changes in albedo and evapotranspiration (Serreze and Barry, 2011), understanding the paleohydrology of Iceland is critical to better understand the past evolution of climate, glaciers, and ecology and to predict future climate and environmental scenarios over the 21st century.
We report Icelandic lake, stream, and soil water isotopes (δ2H and δ18O) measured from samples collected in 2002, 2003, 2004, 2014, 2019, and 2020. Guided by statistical correlation analyses between δ2H; d-excess values; and a suite of climatological, geomorphic, and geographic parameters, we draw the following conclusions.
Lake water isotopes reflect local precipitation, with biases toward the cold season, particularly for lakes with sub-annual residence times, which is consistent with the time of year when most precipitation is delivered. A subset of lakes, which are closed basins, demonstrate δ2Hlake and d-excess values affected by evaporative enrichment. This evaporation likely occurs during the typically open-water summer season when relative humidity is lower, lake water surface temperatures are elevated, and the lake surface is exposed to wind. Lake water isotopes are generally 2H-depleted at higher elevations and at locations farther from the coast, consistently with Rayleigh distillation.
Interannual variabilities of lake water isotopes and d-excess values are consistent with observations that 2019 received less winter snowfall, was relatively dry during the summer, and was characterized by a negative NAO, all contributing to a lower LEL slope and 2H-enriched and low-d-excess lake water relative to 2014 and 2020.
Seasonal variability of lake water isotopes shows that lake surface water is relatively 2H-depleted, with higher d-excess values in February 2019 compared to in September 2019 and 2020, likely due to the predominance of snow over rain in winter months. Additionally, bottom water isotopes reflect winter stratification that is independently recorded in the seasonal temperature and water chemistry cycles of the lakes.
Isotopes and d-excess values of summer surface soil water reflect local precipitation overprinted by evaporative enrichment that can occur throughout the year. The soil water values span the seasonal range of precipitation, suggesting heterogeneity in soil water seasonality. Water isotope measurements from samples collected just tens of meters apart can show large variability, suggesting that additional factors beyond the local climate, especially topography and vegetation, which are inherently related, may be important factors.
The collective dataset optimizes site selection for future studies in Icelandic paleohydrology focusing on changes in moisture balance, precipitation seasonality, and moisture source and provides a process-based framework to guide downcore water isotope proxy interpretations. Specifically, sedimentary records from closed-basin lakes offer the potential to reconstruct Holocene changes in the precipitation–evaporation balance, while sedimentary records from open-basin lakes, particularly those with sub-annual residence times, may provide insight into past changes in moisture source related to winter snowfall variability and atmospheric circulation patterns. At present, the simplest sedimentary records of precipitation would be derived from aquatic-invertebrate (e.g., chironomids) oxygen isotopes given the strong linear relationship observed between lake water and aquatic-invertebrate oxygen isotopes. Future work is needed to constrain the relationship between aquatic plant waxes and their isotopic variability and their relationship to lake water before secure paleohydrological studies can be developed from them. Similarly, given the complexity and uncertainty observed in Icelandic surface soil water isotopes, we urge caution in the application of terrestrial water isotope proxies, such as long-chain n-alkyl lipids, before further calibration studies are conducted.
Water isotopes and associated metadata are archived at the Arctic Data Center (https://doi.org/10.18739/A28W3842T, Harning et al., 2024).
The supplement related to this article is available online at: https://doi.org/10.5194/hess-28-4275-2024-supplement.
ÁG, GHM, JS, YA, and SK funded the research; YA, CRF, JHR, DJH, ÁG, GHM, and JS collected the lake water and soil samples; KBÓ provided Icelandic meteorological data; DJH, JMM, and JHR processed and interpreted the data; DJH led the writing of the paper with contributions from all the co-authors.
The contact author has declared that none of the authors has any competing interests.
Publisher’s note: Copernicus Publications remains neutral with regard to jurisdictional claims made in the text, published maps, institutional affiliations, or any other geographical representation in this paper. While Copernicus Publications makes every effort to include appropriate place names, the final responsibility lies with the authors.
The authors wish to thank Sædís Ólafsdóttir, Jason Briner, Ragna Aðalsteinsdóttir, Pete Langdon, Ian Holmen, Þorsteinn Jónsson, and Sveinbjörn Steinthorsson for their assistance in the field.
This study has been supported by Graduate Students Association (GSA) and Comer Family Foundation funding (awarded to Áslaug Geirsdóttir and Yarrow Axford), the Icelandic Centre for Research (Warm Times–Cold Times grant of excellence, grant no. 022160-002, awarded to Áslaug Geirsdóttir and Gifford H. Miller; ANATILS grant of excellence, grant no. 130775052, awarded to Áslaug Geirsdóttir and Gifford H. Miller; doctoral student grant, grant no. 163431-053, awarded to David J. Harning), the University of Iceland doctoral grant (awarded to Christopher R. Florian and Jonathan H. Raberg), and the National Science Foundation (grant no. ARCSS-1836981, awarded to Gifford H. Miller, Áslaug Geirsdóttir, and Julio Sepúlveda; grant no. EAR-1928303, awarded to Sebastian Kopf and Jamie M. McFarlin).
This paper was edited by Natalie Orlowski and reviewed by two anonymous referees.
Adrian, R., O'Reilly, C. M., Zagarese, H., Baines, S. B., Hessen, D. O., Keller, W., Livingstone, D. M., Sommaruga, R., Straile, D., Van Donk, E., Weyhenmeyer, G. A., and Winder, M.: Lakes as sentinels of climate change, Limnol. Oceanogr., 54, 2283–2297, https://doi.org/10.4319/lo.2009.54.6_part_2.2283, 2009.
Amin, A., Zuecco, G., Geris, J., Schwendenmann, L., McDonnell, J. J., Borga, M., and Penna, D.: Depth distribution of soil water sourced by plants at the global scale: A new direct inference approach, Ecohydrology, 13, e2177, https://doi.org/10.1002/eco.2177, 2020.
Anderson, N. J. and Leng, M. J.: Increased aridity during the early Holocene in West Greenland inferred from stable isotopes in laminated-lake sediments, Quaternary Sci. Rev., 23, 841–849, https://doi.org/10.1016/j.quascirev.2003.06.013, 2004.
Arnalds, O.: Volcanic soils of Iceland, Catena, 56, 3–20, https://doi.org/10.1016/j.catena.2003.10.002, 2004.
Árnason, B.: Groundwater systems in Iceland traced by deuterium, Soc. Sci. Islandica, 42, 1–236, 1976.
Balascio, N. L., D'Andrea, W. J., Bradley, R. S., and Perren, B.: Biogeochemical evidence for hydrologic changes during the Holocene in a lake sediment record from southeast Greenland, Holocene, 23, 1428–1439, https://doi.org/10.1177/0959683613493938, 2013.
Balascio, N. L., D'Andrea, W. J., Gjerde, M., and Bakke, J.: Hydroclimate variability of High Arctic Svalbard during the Holocene inferred from hydrogen isotopes of leaf waxes, Quaternary Sci. Rev., 183, 177–187, https://doi.org/10.1016/j.quascirev.2016.11.036, 2018.
Baldini, L. M., McDermott, F., Foley, A. M., and Baldini, J. U. L.: Spatial variability in the European winter precipitation δ18O-NAO relationship: Implications for reconstructing NAO-mode climate variability in the Holocene, Geogr. Res. Lett., 35, L04709, https://doi.org/10.1029/2007GL032027, 2008.
Bintanja, R. and Selten, F. M.: Future increases in Arctic precipitation linked to local evaporation and sea-ice retreat, Nature, 509, 479–482, https://doi.org/10.1038/nature13259, 2014.
Bintanja, R. and Andry, O.: Towards a rain-dominated Arctic, Nat. Clim. Change, 7, 263–267, https://doi.org/10.1038/nclimate3240, 2017.
Bintanja, R., van der Wiel, K., van der Linden, E. C., Reusen, J., Bogerd, L., Krikken, F., and Selten, F. M.: Strong future increases in Arctic precipitation variability linked to poleward moisture transport, Science Advances, 6, 1–6, https://doi.org/10.1126/sciadv.aax6869 2020.
Bödvarsson, G.: The use of isotopes of hydrogen and oxygen for hydrological purposes in Iceland, Jökull, 12, 49–54, 1962.
Boehrer, B. and Schultze, M.: Stratification of lakes, Rev. Geophys., 46, RG2005, https://doi.org/10.1029/2006RG000210, 2008.
Bowen, G. J., Putman, A., Brooks, J. R., Bowling, D. R., Oerter, E. J., and Good, S. P.: Inferring the source of evaporated waters using stable H and O isotopes, Oecologia, 187, 1025–1039, https://doi.org/10.1007/s00442-018-4192-5, 2018.
Bowen, G. J. and Revenaugh, J.: Interpolating the isotopic composition of modern meteoric precipitation, Water Resour. Res., 39, 1–13, https://doi.org/10.1029/2003WR002086, 2003.
Bush, R. T., Berke, M. A. M., and Jacobson, A. D.: Plant water δD and δ18O of tundra species from west Greenland, Arct. Antarct. Alp. Res., 49, 341–358, https://doi.org/10.1657/AAAR0016-025, 2017.
Caseldine, C., Geirsdóttir, Á., and Langdon, P.: Efstadalsvatn – a multi-proxy study of a Holocene lacustrine sequence from NW Iceland, J. Paleolimnol., 30, 55–73, https://doi.org/10.1023/A:1024781918181, 2003.
Cluett, A. A. and Thomas, E. K.: Resolving combined influences of inflow and evaporation on western Greenland lake water isotopes to inform paleoclimate inferences, J. Paleolimnol., 63, 251–268, https://doi.org/10.1007/s10933-020-00114-4, 2020.
Cook, C. S., Erkkila, B. R., Chakraborty, S., Tipple, B. J., Cerling, T. E., and Ehleringer, J. R.: Stable Isotope Biogeochemistry and Ecology: Laboratory Manual, 173 pp., ISBN 10 1973349086, ISBN 13 978-1973349082, 2017.
Cooper, L. W., Olsen, C. R., Solomon, D. K., Larsen, I. L., Cook, R. B., and Grebmeier, J. M.: Stable isotopes of oxygen and natural and fallout radionuclides used for tracing runoff during snowmelt in an arctic watershed, Water Resour. Res., 27, 2171–2179, https://doi.org/10.1029/91WR01243, 1991.
Corcoran, M. C., Thomas, E. K., and Morrill, C.: Using a paired chironomid δ18O and aquatic leaf wax δ2H approach to reconstruct seasonality on western Greenland during the Holocene, Paleoceanography and Paleoclimatology, 36, e2020PA004169, https://doi.org/10.1029/2020PA004169, 2021.
Cowling, O. C., Thomas, E. K., Svendsen, J. I., Mangerud, J., Haflidason, H., Regnéll, C., and Brendyren, J.: Western Siberia experience rapid shifts in moisture source and summer water balance during the last deglaciation and early Holocene, J. Quaternary Sci., 37, 790–804, https://doi.org/10.1002/jqs.3386, 2022.
Craig, H.: Isotopic variations in meteoric waters, Science, 133, 1702–1703, https://doi.org/10.1126/science.133.3465.1702, 1961.
Crochet, P., Jóhannesson, T., Jónsson, T., Sigurðsson, O., Björnsson, H., Pálsson, F., and Barstad, I.: Estimating the spatial distribution of precipitation in Iceland using a linear model of orographic precipitation, Journal of Hydrometerology, 8, 1285–1306, https://doi.org/10.1175/2007JHM795.1, 2007.
Curtin, L., D'Andrea, W. J., Balascio, N., Pugsley, G., de Wet, G., and Bradley, R.: Holocene and Last Interglacial climate of the Faroe Islands from sedimentary plant wax hydrogen and carbon isotopes, Quaternary Sci. Rev., 223, 105930, https://doi.org/10.1016/j.quascirev.2019.105930, 2019.
Dansgaard, W.: Stable isotopes in precipitation, Tellus, 16, 436–468, https://doi.org/10.1111/j.2153-3490.1964.tb00181.x, 1964.
Dion-Kirschner, H., McFarlin, J. M., Masterson, A. L., Axford, Y., and Osburn, M. R.: Modern constraints on the sources and climate signals recorded by sedimentary plant waxes in west Greenland, Geochim. Cosmochim. Ac., 286, 336–354, https://doi.org/10.1016/j.gca.2020.07.027, 2020.
Douville, H., Raghavan, K., Renwick, J., Allan, R. P., Arias, P. A., Barlow, M., Cerezo-Mota, R., Cherchi, A., Gan, T. Y., Gergis, J., Jiang, D., Khan, A., Pokam Mba, W., Rosenfeld, D., Tierney, J., and Zolina, O.: Water Cycle Changes. In Climate Change 2021: The Physical Science Basis. Contribution of Working Group I to the Sixth Assessment Report of the Intergovernmental Panel on Climate Change, edited by: Masson-Delmotte, V., Zhai, P., Pirani, A., Connors, S. L., Péan, C., Berger, S., Caud, N., Chen, Y., Goldfarb, L., Gomis, M. I., Huang, M., Leitzell, K., Lonnoy, E., Matthews, J. B. R., Maycock, T. K., Waterfield, T., Yelekçi, O., Yu, R., and Zhou, B., Cambridge University Press, Cambridge, United Kingdom and New York, NY, USA, 1055–1210, 2021.
Eensalu, M., Nelson, D. B., Buczynska, A., Rach, O., Klein, E. S., Dodd, J. P., Poska, A., and Stansell, N. D.: Hydrogen isotope biogeochemistry of plant waxes in paired lake catchments, Org. Geochem., 184, 104674, https://doi.org/10.1016/j.orggeochem.2023.104674, 2023.
Einarsson, M. Á.: Climate of Iceland – World Survey of Climatology, Vol. 15, Climate of the Oceans, edited by: van Loon, H., Elsevier, Amsterdam, 673–697, 1984.
Epstein, S. and Mayeda, T.: Variation of O18 content of waters from natural sources, Geochim. Cosmochim. Ac., 4, 213–224, https://doi.org/10.1016/0016-7037(53)90051-9, 1953.
Feng, X., Lauder, A. M., Posmentier, E. S., Kopec, B. G., and Virginia, R. A.: Evaporation and transport of water isotopologues from Greenland lakes: The lake size effect, Quaternary Sci. Rev., 131, 302–315, https://doi.org/10.1016/j.quascirev.2015.07.029, 2016.
Florian, C. R.: Multi-proxy reconstructions of Holocene environmental change and catchment biogeochemistry using algal pigments and stable isotopes preserved in lake sediment from Baffin Island and Iceland, PhD thesis, University of Colorado Boulder and University of Iceland, p. 141, https://scholar.colorado.edu/concern/graduate_thesis_or_dissertations/rb68xb91b (last access: 1 January 2024), 2016.
Friedman, I.: Sigurgeirsson Th., and Gardarsson, Ö.: Deuterium in Iceland waters, Geochim. Cosmochim. Ac., 27, 553–561, https://doi.org/10.1016/0016-7037(63)90012-7, 1963.
Gat, J. R.: Oxygen and hydrogen isotopes in the hydrologic cycle, Annu. Rev. Earth Pl. Sc., 24, 225–262, https://doi.org/10.1146/annurev.earth.24.1.225, 1996.
Geirsdóttir, Á., Miller, G. H., Larsen, D. J., and Ólafsdóttir, S.: Abrupt Holocene climate transitions in the northern North Atlantic region recorded by synchronized lacustrine records in Iceland, Quaternary Sci. Rev., 70, 48–62, https://doi.org/10.1016/j.quascirev.2013.03.010, 2013.
Geirsdóttir, Á., Harning, D. J., Miller, G. H., Andrews, J. T., Zhong, Y., and Caseldine, C.: Holocene history of landscape instability in Iceland: Can we deconvolve the impacts of climate, volcanism and human activity?, Quaternary Sci. Rev., 249, 106633, https://doi.org/10.1016/j.quascirev.2020.106633, 2020.
Geris, J., Tetzlaff, D., McDonnell, J., and Soulsby, C.: The relative role of soil type and tree cover on water storage and transmission in northern headwater catchments, Hydrol. Process., 29, 1844–1860, https://doi.org/10.1002/hyp.10289, 2015a.
Geris, J., Tetzlaff, D., McDonnell, J., Anderson, J., Paton, G., and Soulsby, C.: Ecohydrological separation in wet, low energy northern environments? A preliminary assessment using different soil water extraction techniques, Hydrol. Process., 29, 5139–5152, https://doi.org/10.1002/hyp.10603, 2015b.
Gibson, J. J. and Edwards, T. W. D.: Regional water balance trends and evaporation-transpiration partitioning from a stable isotope survey of lakes in northern Canada, Global Biogeochem. Cy., 16, 1011–1014, https://doi.org/10.1029/2001GB001839, 2002.
Gibson, J. J., Prepas, E. E., and McEachern, P.: Quantitative comparison of lake throughflow, residency, and catchment runoff using stable isotopes: Modelling and results from a regional survey of Boreal lakes, J. Hydrol., 262, 128–144, https://doi.org/10.1016/S0022-1694(02)00022-7, 2002.
Gibson, J. J., Birks, S. J., and Yi, Y.: Stable isotope mass balance of lakes: a contemporary perspective, Quaternary Sci. Rev., 131, 316–328, https://doi.org/10.1016/j.quascirev.2015.04.013, 2016.
Gonfiantini, R., Wassenaar, L. I., and Araguas-Araguas, L. J.: Stable isotope fractionations in the evaporation of water: The wind effect, Hydrol. Process., 34, 3596–3607, https://doi.org/10.1002/hyp.13804, 2020.
Google Earth: 12/13/2015, https://earth.google.com/web/date, last access: 2 January 2023.
Gorbey, D. B., Thomas, E. K., Sauer, P. E., Raynolds, M. K., Miller, G. H., Corcoran, M. C., Cowling, O. C., Crump, S. E., Lovell, K., and Raberg, J. H.: Modern eastern Canadian Arctic lake water isotopes exhibit latitudinal patterns in inflow seasonality and minimal evaporative enrichment, Paleoceanography and Paleoclimatology, 37, 1–19, https://doi.org/10.1029/2021PA004384, 2022.
Hanna, E., Jónsson, T., and Box, J. E.: An analysis of Icelandic climate since the nineteenth century, Int. J. Climatol., 24, 1193–1210, https://doi.org/10.1002/joc.1051, 2004.
Hanna, E., Jónsson, T., Ólafsson, J., and Valdimarsson, H.: Icelandic coastal sea surface temperature records constructed: Putting the pulse on air-sea-climate interactions in the Northern North Atlantic. Part 1: Comparison with HadISST1 open-ocean surface temperatures and preliminary analysis of long-term patterns and anomalies of SSTs around Iceland, J. Climate, 19, 5652–5667, https://doi.org/10.1175/JCLI3933.1, 2006.
Harning, D., Raberg, J., McFarlin, J., Axford, Y., Florian, C., Ólafsdóttir, K., Kopf, S., Sepúlveda, J., Miller, G., and Geirsdóttir, Á.: Lake, stream, and soil water isotopes in Iceland (2002–2004, 2014, 2019–2020), Arctic Data Center [data set], https://doi.org/10.18739/A28W3842T, 2024.
Hollister, K. V., Thomas, E. K., Raynolds, M. K., Bültmann, H., Raberg, J. H., Miller, G. H., and Sepúlveda, J.: Aquatic and terrestrial plant contributions to sedimentary plant waxes in a modern Arctic lake setting, J. Geophys. Res.-Biogeo., 127, e2022JG006903, https://doi.org/10.1029/2022JG006903, 2022.
Holtvoeth, J., Whiteside, J. H., Engels, S., Freitas, F. S., Grice, K., Greenwood, P., Johnson, S., Kendall, I., Lengger, S. K., Lücke, A., Mayr, C., Naafs, B. D. A., Rohrssen, M., and Sepúlveda, J.: The paleolimnologist's guide to compound-specific stable isotope analysis – An introduction to principles and applications of CSIA for Quaternary lake sediments, Quaternary Sci. Rev., 207, 101–133, https://doi.org/10.1016/j.quascirev.2019.01.001, 2019.
Hurrell, J. W., Kushnir, Y., Ottersen, G., and Visbeck, M.: An overview of the North Atlantic Oscillation, Geophysical Monograph, 134, 1–35, https://doi.org/10.1029/134GM01, 2003.
IAEA/WMO: Global network of isotopes in precipitation. The GNIP Database, https://nucleus.iaea.org/wiser (last access: 1 January 2024), 2015.
Icelandic Met Office (IMO): Climatological data, https://en.vedur.is/climatology/data/ (last access: 1 January 2024), 2023.
Jonsson, C. E., Leng, M. J., Rosqvist, G. C., Seibert, J., and Arrowsmith, C.: Stable oxygen and hydrogen isotopes in sub-Arctic lake waters from northern Sweden, J. Hydrol., 376, 143–151, https://doi.org/10.1016/j.jhydrol.2009.07.021, 2009.
Jouzel, J., Hoffmann, G., Koster, R. D., and Masson, V.: Water isotopes in precipitation: data/model comparison for present-day and past climates, Quaternary Sci. Rev., 19, 363–379, https://doi.org/10.1016/S0277-3791(99)00069-4, 2000.
Kjellman, S. E., Schomacker, A., Thomas, E. K., Håkansson, L., Duboscq, S., Cluett, A. A., Farnsworth, W. R., Allaart, L., Cowling, O. C., McKay, N. P., Brynjólfsson, S., and Ingólfsson, Ó.: Holocene precipitation seasonality in northern Svalbard: Influence of sea ice and regional ocean surface conditions, Quaternary Sci. Rev., 240, 106388, https://doi.org/10.1016/j.quascirev.2020.106388, 2020.
Kjellman, S. E., Thomas, E. K., and Schomacker, A.: Arctic and sub-Arctic lake water δ2H and δ18O along a coastal-inland transect: Implications for interpreting water isotope proxy records, J. Hydrol., 607, 127556, https://doi.org/10.1016/j.jhydrol.2022.127556, 2022.
Kopec, B. G., Feng, X., Posmentier, E. S., Chipman, J. W., and Virginia, R. A.: Use of principal component analysis to extract environmental information from lake water isotopic compositions, Limnol. Oceanogr., 63, 1340–1354, https://doi.org/10.1002/lno.10776, 2018.
Konecky, B. L., McKay, N. P., Falster, G. M., Stevenson, S. L., Fischer, M. J., Atwood, A. R., Thompson, D. M., Jones, M. D., Tyler, J. J., DeLong, K. L., Martrat, B., Thomas, E. K., Conroy, J. L., Dee, S. G., Jonkers, L., Churakova (Sidorova), O. V., Kern, Z., Opel, T., Porter, T. J., Sayani, H. R., Skrzypek, G., and Iso2k Project Members: Globally coherent water cycle response to temperature change during the past two millennia, Nat. Geosci., 16, 997–1004, https://doi.org/10.1038/s41561-023-01291-3, 2023.
Leng, M. J. and Anderson, N. J.: Isotopic variation in modern lake waters from western Greenland, Holocene, 13, 605–611, https://doi.org/10.1191/0959683603hl620rr, 2003.
Linderholm, H. W., Nicolle, M., Francus, P., Gajewski, K., Helama, S., Korhola, A., Solomina, O., Yu, Z., Zhang, P., D'Andrea, W. J., Debret, M., Divine, D. V., Gunnarson, B. E., Loader, N. J., Massei, N., Seftigen, K., Thomas, E. K., Werner, J., Andersson, S., Berntsson, A., Luoto, T. P., Nevalainen, L., Saarni, S., and Väliranta, M.: Arctic hydroclimate variability during the last 2000 years: current understanding and research challenges, Clim. Past, 14, 473–514, https://doi.org/10.5194/cp-14-473-2018, 2018.
McFarlin, J. M., Axford, Y., Masterson, A. L., and Osburn, M. R.: Calibration of modern sedimentary δ2H plant wax-water relationships in Greenland lakes, Quaternary Sci. Rev., 225, 105978, https://doi.org/10.1016/j.quascirev.2019.105978, 2019.
McFarlin, J. M., Axford, Y., Kusch, S., Masterson, A. L., Lasher, G. E., and Osburn, M. R.: Aquatic plant wax hydrogen and carbon isotopes in Greenland lakes record shifts in methane cycling during past Holocene warming, Science Advances, 9, 1–12, https://doi.org/10.1126/sciadv.adh9704, 2023.
Moossen, H., Bendle, J., Seki, O., Quillmann, U., and Kawamura, K.: North Atlantic Holocene climate evolution recorded by high-resolution terrestrial and marine biomarker records, Quaternary Sci. Rev., 129, 111–127, https://doi.org/10.1016/j.quascirev.2015.10.013, 2015.
Muhic, F., Ala-Aho, P., Noor, K., Welker, J. M., Klöve, B., and Marttila, H.: Flushing or mixing? Stable isotopes reveal differences in arctic forest and peatland soil water seasonality, Hydrol. Process., 37, e14811, https://doi.org/10.1002/hyp.14811, 2023.
NOAA National Centers for Environmental Information: North Atlantic Oscillation (NAO), https://www.ncei.noaa.gov/access/monitoring/nao/ (last access: 1 January 2024), 2023.
Olsen, J., Anderson, N. J., and Knudsen, M. F.: Variability of the North Atlantic Oscillation over the past 5,200 years, Nat. Geosci., 5, 1–14, https://doi.org/10.1038/ngeo1589, 2012.
R Core Team: R: A Language and Environment for Statistical Computing. R Foundation for Statistical Computing, Vienna, Austria, https://www.R-project.org/ (last access: 1 January 2024), 2022.
Raberg, J., Harning, D., Geirsdóttir, Á., Sepúlveda, J., and Gifford Miller, G. H.: Soil and lake water temperatures of Iceland (2019–2021), Arctic Data Center [data set], https://doi.org/10.18739/A2XP6V46R, 2021.
Raberg, J., Harning, D., Geirsdóttir, Á., Sepúlveda, J., and Gifford Miller, G. H.: Water chemistry profiles of lakes in Iceland (2019–2021), Arctic Data Center [data set], https://doi.org/10.18739/A26688K7F, 2023.
Raberg, J. H., Crump, S. E., de Wet, G., Harning, D. J., Miller, G. H., Geirsdóttir, Á., and Sepúlveda, J.: BrGDGT lipids reflect summer soil temperature and seasonal soil water chemistry, Geochim. Cosmochim. Ac., 369, 111–125, https://doi.org/10.1016/j.gca.2024.01.034, 2024.
Raberg, J. H., Harning, D. J., Crump, S. E., de Wet, G., Blumm, A., Kopf, S., Geirsdóttir, Á., Miller, G. H., and Sepúlveda, J.: Revised fractional abundances and warm-season temperatures substantially improve brGDGT calibrations in lake sediments, Biogeosciences, 18, 3579–3603, https://doi.org/10.5194/bg-18-3579-2021, 2021.
Rach, O., Kahmen, A., Brauer, A., and Sachse, D.: A dual-biomarker approach for quantification of changes in relative humidity from sedimentary lipid D∕H ratios, Clim. Past, 13, 741–757, https://doi.org/10.5194/cp-13-741-2017, 2017.
Sachse, D., Billault, I., Bowen, G. J., Chikaraishi, Y., Dawson, T. E., Feakins, S. J., Freeman, K. H., Magill, C. R., McInerney, M. A., van der Meer, M. T. J., Polissar, P., Robins, R. J., Sachs, J. P., Schmidt, H.-L., Sessions, A. L., White, J. W. C., West, J. B., and Kahmen, A.: Molecular paleohydrology: Interpreting the hydrogen-isotopic composition of lipid biomarkers from photosynthesizing organisms, Annu. Rev. Earth Pl. Sc., 40, 221–249, https://doi.org/10.1146/annurev-earth-042711-105535, 2012.
Serreze, M. C., Barrett, A. P., Slater, A. G., Woodgate, R. A., Aagaard, K., Lammers, R. B., Steele, M., Moritz, R., Meredith, M., and Lee, C. M.: The large-scale freshwater cycle of the Arctic, J. Geophys. Res.-Oceans, 111, 1–19, https://doi.org/10.1029/2005JC003424, 2006.
Serreze, M. C. and Barry, R. G.: Processes and impacts of Arctic amplification: A research synthesis, Global Planet. Change, 77, 85–96, https://doi.org/10.1016/j.gloplacha.2011.03.004, 2011.
Sodemann, H., Masson-Delmotte, V., Schwierz, C., Vinther, B. M., and Wernli, H.: Interannual variability of Greenland winter precipitation sources: 2. Effects of North Atlantic Oscillation variability on stable isotopes in precipitation, J. Geophys. Res., 113, 1–21, https://doi.org/10.1029/2007JD009416, 2008.
Sprenger, M., Tetzlaff, D., and Soulsby, C.: Soil water stable isotopes reveal evaporation dynamics at the soil–plant–atmosphere interface of the critical zone, Hydrol. Earth Syst. Sci., 21, 3839–3858, https://doi.org/10.5194/hess-21-3839-2017, 2017.
Steen-Larsen, H. C., Sveinbjörnsdóttir, A. E., Jonsson, Th., Ritter, F., Bonne, J.-L., Masson-Delmotte, V., Sodemann, H., Blunier, T., Dahl-Jensen, D., and Vinther, B. M.: Moisture sources and synoptic to seasonal variability of North Atlantic water vapor isotopic composition, J. Geophys. Res.-Atmos., 120, 5757–5774, https://doi.org/10.1002/2015JD023234, 2015.
Stefánsson, A., Arnórsson, S., Sveinbjörnsdóttir, Á. E., Heinemeier, J., and Kristmannsdóttir, H.: Isotope (δD, δ18O, 3H, δ13C, 14C) and chemical (B, Cl) constrains on water origin, mixing, water-rock interaction and age of low-temperature geothermal water, Appl. Geochem., 108, 104380, https://doi.org/10.1016/j.apgeochem.2019.104380, 2019.
Stefánsson, U.: North Icelandic Waters, Rit Fiskideildar, 3, 1–265, 1962.
Sundqvist, H. S., Kaufman, D. S., McKay, N. P., Balascio, N. L., Briner, J. P., Cwynar, L. C., Sejrup, H. P., Seppä, H., Subetto, D. A., Andrews, J. T., Axford, Y., Bakke, J., Birks, H. J. B., Brooks, S. J., de Vernal, A., Jennings, A. E., Ljungqvist, F. C., Rühland, K. M., Saenger, C., Smol, J. P., and Viau, A. E.: Arctic Holocene proxy climate database – new approaches to assessing geochronological accuracy and encoding climate variables, Clim. Past, 10, 1605–1631, https://doi.org/10.5194/cp-10-1605-2014, 2014.
Tetzlaff, D., Birkel, C., Dick, J., Geris, J., and Soulsby, C.: Storage dynamics in hydropedological units control hillslope connectivity, runoff generation, and the evolution of catchment transit time distributions, Water Resour. Res., 50, 969–985, https://doi.org/10.1002/2013WR014147, 2014.
Tetzlaff, D., Piovano, T., Ala-Aho, P., Smith, A., Carey, S. K., Marsh, P., Wookey, P. A., Street, L. E., and Soulsby, C.: Using stable isotopes to estimate travel times in a data-sparse Arctic catchment: Challenges and possible solutions, Hydrol. Process., 32, 1936–1952, https://doi.org/10.1002/hyp.13146, 2018.
Thomas, E. K., Hollister, K. V., Cluett, A. A., and Corcoran, M. C.: Reconstructing Arctic precipitation seasonality using aquatic leaf wax δ2H in lakes with contrasting residence times, Paleoceanography and Paleoclimatology, 35, 1–19, https://doi.org/10.1029/2020PA003886, 2020.
Throckmorton, H. M., Newman, B. D., Heikoop, J. M., Perkins, G. B., Feng, X., Graham, D. E., O`Malley D., Vesselinov, V. V., Young, J., Wullschleger, S. D., and Wilson, C. J.: Active layer hydrology in an arctic tundra ecosystem: quantifying water sources and cycling using water stable isotopes, Hydrol. Process., 30, 4972–4986, https://doi.org/10.1002/hyp.10883, 2016.
van der Bilt, W. G. M., D'Andrea, W. J., Oppedal, L. T., Bakke, J., Bjune, A. E., and Zwier, M.: Stable Southern Hemisphere westerly winds throughout the Holocene until intensification in the last two millennia, Commun. Earth Environ., 3, 186, https://doi.org/10.1038/s43247-022-00512-8, 2022.
Vaughn, B. H., White, J. W. C., Delmotte, M., Trolier, M., Cattani, O., and Stievenard, M.: An automated system for hydogen isotope analysis of water, Chem. Geol., 152, 309–319, https://doi.org/10.1016/S0009-2541(98)00117-X, 1998.
Verbruggen, F., Heiri, O., Reichart, G. J., Blaga, C., and Lotter, A. F.: Stable oxygen isotopes in chironomid and cladoceran remains as indicates for lake-water δ18O, Limnol. Oceanogr., 56, 2071–2079, https://doi.org/10.4319/lo.2011.56.6.2071, 2011.
Wei, T. and Simko, V.: R package “corrplot”: Visualization of a Correlation Matrix (Version 0.92), GitHub [code], https://github.com/taiyun/corrplot (last access: 1 January 2024), 2021.
Wooller, M., Wang, Y., and Axford, Y.: A multiple stable isotope record of Late Quaternary limnological changes and chironomid paleoecology from northeastern Iceland, J. Paleolimnology, 40, 63–77, https://doi.org/10.1007/s10933-007-9144-8, 2008.