the Creative Commons Attribution 4.0 License.
the Creative Commons Attribution 4.0 License.
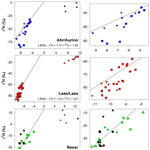
A comparative study of plant water extraction methods for isotopic analyses: Scholander-type pressure chamber vs. cryogenic vacuum distillation
Anam Amin
Jay Frentress
Michael Engel
Chiara Marchina
Tommaso Anfodillo
Marco Borga
Vinicio Carraro
Francesca Scandellari
Massimo Tagliavini
Damiano Zanotelli
Francesco Comiti
Daniele Penna
Recent tracer-based studies using stable isotopes of hydrogen and oxygen showed that different methods for extracting water from plant tissues can return different isotopic compositions due to the presence of organic compounds and because they extract different plant water domains. One of the most used methods to extract plant water is the cryogenic vacuum distillation (CVD), which tends to extract total plant water. Conversely, the Scholander-type pressure chamber (SPC), which is commonly used by tree physiologists to measure water potential in plant tissues and determine plant water stress, is expected to extract only the more mobile plant water (i.e., xylem and inter-cellular water). However, only few studies reported the application of SPC to extract plant water for isotopic analyses, and therefore, inter-method comparisons between SPC and CVD are of great value.
In this work, we analyzed the variability in the isotopic composition of plant water extracted by SPC and CVD, also considering the potential variability in the isotopic signature of the plant water extracted by CVD from various tissues (i.e., leaves, twig without bark, twig with bark, twig close to the trunk of the tree, and wood core) and from different plant species (i.e., alder, apple, chestnut, and beech). The extraction of plant water by SPC is simple, can be carried out in the field, and it does not require specific laboratory work as in the case of CVD. However, the main limitation of SPC is the very small water volume that can be extracted from the lignified twigs under water stress conditions compared to CVD.
Our results indicated that plant water extracted by SPC and CVD were significantly different. The difference in the isotopic composition obtained by the two extraction methods was smaller in the beech samples compared to alder, apple, and chestnut samples. The isotopic signature of alder, apple, and chestnut plant water extracted by SPC was more enriched in δ2H and δ18O, respectively, than the samples obtained by CVD. We conclude that plant water extraction by SPC is not an alternative for CVD as SPC mostly extracts the mobile plant water, whereas CVD tends to retrieve all water stored in the sampled tissue from both living and dead cells. However, studies aiming to quantify the relative contribution of the soil water sources to transpiration should rely more on the isotopic composition of xylem water (which is theoretically sampled by SPC) than the isotopic composition of total plant water (sampled by CVD), which also contains a fraction of water that could be stored in plant tissues for a longer time.
- Article
(5175 KB) - Full-text XML
- BibTeX
- EndNote
Stable isotopes in the water molecule (2H and 18O) have been extensively used as environmental tracers in hydrological studies to track water fluxes and estimate water flow pathways, mean residence times, and water storage (e.g., Dansgaard, 1953; Craig, 1961; Klaus and McDonnell, 2013). The development of low-cost and easy-to-use spectroscopic techniques for the collection and isotopic analysis of water samples at a high temporal resolution (e.g., Kerstel et al., 1999; Penna et al., 2010; von Freyberg et al., 2017) stimulated the application of stable isotopes to investigate water transfer in the soil–plant–atmosphere continuum (Brooks et al., 2010; McDonnell, 2014). An increasing number of studies have been recently conducted to better understand water dynamics, such as water uptake and evapotranspiration partitioning, in the soil–plant–low atmosphere continuum in different climates and in both natural (e.g., Allen et al., 2019; Dubbert et al., 2019; Engel et al., 2022; Liu et al., 2019a; Oerter et al., 2019; Qiu et al., 2019) and managed (agricultural and agroforest) (e.g., Aguzzoni et al., 2022; Liu et al., 2019b; Quade et al., 2019; Zhang et al., 2019; Penna et al., 2020) environments. Despite the rapid increase of the number of studies based on the stable isotope approach, only a small fraction of them compared two or more plant water extraction techniques (e.g., Millar et al., 2018; Fischer et al., 2019; Barbeta et al., 2022).
Ecohydrological studies relying on the isotopic signature of plant water require sampling methods that extract water representative of transpiration while not altering the original isotopic composition of the plant material. This is still a critical aspect because a standardized methodology for isotope-based ecohydrological studies has not been defined yet (Penna et al., 2018). Indeed, several techniques for the extraction of plant water exist, such as in situ direct vapor equilibration (Sprenger et al., 2015; Volkmann et al., 2016; Marshall et al., 2020), microwave extraction (Munksgaard et al., 2014), cryogenic vacuum distillation (Koeniger et al., 2011; Orlowski et al., 2013, 2016a), centrifugation (Peters and Yakir, 2008), and high-pressure mechanical squeezing (Böttcher et al., 1997). In addition, xylem water can be extracted by a syringe with a needle inserted in a pre-drilled hole in the stem, exploiting the positive inner pressure present in some tree species (Zhao et al., 2016), or by a cavitron flow-rotor (Barbeta et al., 2022). Cryogenic vacuum distillation (abbreviated to CVD thereinafter) is also widely applied (Orlowski et al., 2018; Amin et al., 2020). During CVD, the soil or plant material is heated in a tube under a specified vacuum to evaporate the sample water that afterwards is frozen and collected in a cryogenic trap (Koeniger et al., 2011; Orlowski et al., 2013). As such, this technique extracts the entire volume of water from plant tissues, including water within cell walls (Millar et al., 2018). This volume may include water that underwent fractionation processes and/or water having different ages and stored in dead and living cells for days or weeks (Sprenger et al., 2019), so it is not only water that is transported at the time of the sampling. This might be a serious limitation in ecohydrological and physiological studies aiming to understand water sources for plant transpiration. Indeed, the isotopic composition of water stored in plant tissues for a long time is possibly different from that of xylem water. Moreover, experimental evidence showed that different techniques might return different isotopic values due to intrinsic methodological differences (Beyer and Penna, 2021). CVD, in the case of soil water, was shown to reveal large differences in the isotopic composition of water extracted from soil samples by different laboratories even though strictly consistent procedures were applied (Orlowski et al., 2018). These authors also observed no clear trends in the results and differences depended on the interplay of multiple factors, such as soil type and properties, soil water content, system setup, extraction efficiency, extraction system leaks, and each laboratory’s internal accuracy.
Recently, Millar et al. (2018) performed a thorough comparison of six plant water extraction techniques (i.e., direct vapor equilibration, microwave extraction, two versions of CVD, centrifugation, and high-pressure mechanical squeezing) based on four plant portions of spring wheat (Triticum aestivum). The authors found marked differences among the measured isotopic compositions of plant water, with the CVD systems and the high-pressure mechanical squeezing producing waters more depleted in heavy isotopes compared to the other techniques. Particularly, Millar et al. (2018) associated the differences in the isotopic compositions of plant water to the ability of each extraction system to access different plant water domains. The authors argued that CVD, microwave extraction, centrifugation, and high-pressure mechanical squeezing could access all plant water domains (i.e., the more mobile xylem water and inter-cellular water, and the less mobile intra-cellular, cell wall, and organelle-constrained water), whereas direct vapor equilibration could only extract the mobile xylem and inter-cellular water. Millar et al. (2018) concluded that, in terms of the limited co-extraction of organic compounds and speed of sample throughput, the direct vapor equilibration outperformed CVD.
Fischer et al. (2019) proposed and described various low-tech plant water sampling and extraction techniques, and compared them to the CVD developed by Koeniger et al. (2011) for different plant species. Fischer et al. (2019) found that the new methods produced consistent and comparable results to those provided by CVD. However, these authors, due to the limited amount of plant material, could not assess the water domains accessed by the different methods for each plant type. Fischer et al. (2019) also showed that other factors, such as appropriate transport and storage of the samples from the field site to the laboratory, fast sample processing, and efficient workflows, significantly influenced the accuracy and the precision of the measured isotopic composition.
Comparing different techniques for plant water extraction, understanding which water domain each method accesses, and whether isotopic fractionation occurs during the extraction process are becoming increasingly important, particularly when isotopic differences between plant water and the respective potential water sources used for transpiration are observed. Indeed, Barbeta et al. (2019) found that isotopic fractionation resulting in an unexpected depleted δ2H of xylem water complicated the identification and quantification of the water sources used by beech trees in a temperate forest in France. These authors recommended that future research should investigate the physico–chemical fractionation processes occurring in the unsaturated zone and improve the understanding of the isotopic dynamics of water stored within the plant tissues. If plant water domains have distinct isotopic signatures, new techniques should be developed with the aim of extracting the target plant water domain. More recently, studies by Chen et al. (2020) and Barbeta et al. (2022) observed that δ2H of stem water extracted by CVD had a significant depletion compared to δ2H of xylem water (however, such large difference was not found for δ18O), but there was no significant δ2H offset between xylem and source waters. Barbeta et al. (2022) commented that factors leading to these δ2H offsets are still unclear. Indeed, while Chen et al. (2020) proposed that the offset was due to the H exchange between organically bound deuterium in the wood material and liquid water during the CVD extraction, other studies (e.g., Zhao et al., 2016; Barbeta et al., 2020) suggested a possible effect of aquaporin- or surface-mediated within-stem water isotope heterogeneity on the δ2H offset. This δ2H bias in the CVD extraction can significantly affect the plant water source identification (Allen and Kirchner, 2022; Barbeta et al., 2022). The effect of the δ2H bias on the inference of the plant water sources can be substantial when the isotopic differences among the end members are small, whereas this effect is less marked when there is a large difference among the isotopic compositions of the end members (Allen and Kirchner, 2022).
However, all the comparative studies mentioned above have not considered ecophysiological-based methods that tree physiologists usually adopt to measure leaf water potential and determine plant water stress (e.g., Scholander, 1966; Meiri et al., 1975; Grossiord et al., 2017; Bowling et al., 2017). One of these methods, namely, the Scholander-type pressure chamber (abbreviated to SPC thereinafter) is based on an external pressure to retrieve the mobile water transported within the xylem conduits to measure the plant water potential. Although SPC is widely used in plant water relations studies to measure plant water potential, it is not commonly applied to collect the extracted water for isotopic analyses. We have found only four published studies (Ellsworth and Williams, 2007; Penna et al., 2013; Geißler et al., 2019; Magh et al., 2020) that used SPC to extract plant water for isotopic analysis. One of them, Geißler et al. (2019), made a simple comparison between δ18O of water extracted by SPC and CVD in stem water samples collected from Acacia mellifera. Samples were 10 cm long and lignified, and, for SPC extraction, the authors removed leaves, bark, and green tissues to avoid contamination with phloem. Overall, Geißler et al. (2019) found no significant difference in the isotopic composition of the plant water extracted by the two methods. However, this analysis was based on the comparison of six samples and one plant species only, and more robust comparative tests are missing. Therefore, the assessment of potential differences in isotope data retrieved by using SPC and the CVD extraction techniques based on a larger number of samples and different plant species is still lacking.
In this study, we assumed that SPC extracts relatively mobile plant water only, in contrast to CVD which accesses all plant water domains (Millar et al., 2018) and water potentially fractionated. Given that the relatively mobile plant water might have a different age and a different isotopic composition compared to the less mobile water domain (Sprenger et al., 2019), we hypothesized that SPC and CVD return significant differences in the isotopic composition of the extracted plant water. Therefore, our specific objectives were to: (i) quantify the differences in the isotopic composition of plant water extracted by the two techniques and (ii) assess how differences in the isotopic composition are related to plant species or plant tissue type used for CVD.
2.1 Ahr/Aurino
Samples from gray alder trees (Alnus incana) were taken at two sites in the riparian area of the Ahr/Aurino River in the eastern Italian Alps (Fig. 1). The study site is located at about 882 m above sea level (a.s.l.) in the lower valley where the typical valley form is U-shaped. The catchment is mostly composed of metamorphic (gneiss, mica schists) and magmatic (tonalite) rocks. The median diameter of sediment in the upper meter of soil in the former floodplain ranges from 0.3 to 0.5 mm (Andreoli et al., 2020). The climate is cold temperate with an average annual air temperature of about 7.7 ∘C (period 1992–2018) and an average yearly precipitation amount of about 821 mm yr−1 (period 1972–2018). The Ahr/Aurino River regime is nivo-glacial (the glacierized area is about 4 %). Riparian vegetation mainly consists of mature (at the upstream site in Fig. 1) and young patches (at the downstream site) of gray alder with a thick tall herb (Rubus caesius, Glechoma hederacea and Urtica dioica, Sambucus nigra shrubs, and the vine Humulus lupulus). Gravel mining activities in the 1950s to the 1980s resulted in riverbed incision and a floodplain being disconnected from its channel (Campana et al., 2014).
The sampling campaign was carried out on 7 June 2017 during a period of prolonged water deficit. Due to logistic issues and to collect samples when the transpiration fluxes were close to their minimum, plant water was collected after sunset from four alder trees (two at the downstream and two at the upstream site) in the Ahr/Aurino study area. Samples for water extraction by SPC and CVD were taken from the same position in the trees. More details on the sampling sites and on the methodology can be found in Engel et al. (2022).
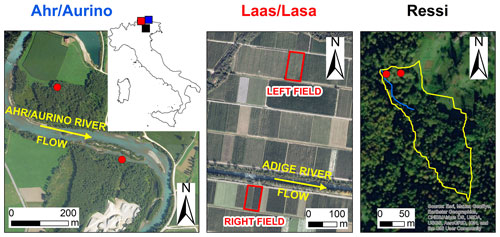
Figure 1Aerial photos of the study sites and location in northern Italy (blue: Ahr/Aurino; red: Laas/Lasa; black: Ressi). Red dots in Ahr/Aurino and Ressi indicate the approximate position of the sampled trees. In Ressi, the blue and the yellow solid lines mark the stream network and the catchment divide, respectively. Sources of aerial photos: © Autonomous Province of Bolzano-South Tyrol (study sites: Ahr/Aurino and Lasa); Esri, “World Imagery” [basemap], scale not given, “World Imagery”, 14 August 2020, http://www.arcgis.com/home/item.html?id=10df2279f9684e4a9f6a7f08febac2a9, (last access: 1 September 2020) (study site: Ressi). The maps were made using Esri ArcGIS 10.7.1.
2.2 Laas/Lasa
Samples from cultivated apple trees (Malus domestica, cv. “Pinova” grafted on “M9” rootstock) were collected in two apple orchards in the Laas/Lasa (Vinschgau/Val Venosta region, South Tyrol; Fig. 1). The orchards are located at about 860 m a.s.l. on the right and left side of the river Etsch/Adige, with different distances from the river (50 vs. 450 m, respectively). Within each orchard, a plot of about 400 m2 was selected for sampling. The average annual precipitation recorded at the Laas/Lasa weather station (874 m a.s.l., operated by the Hydrographic Office of the Autonomous Province of Bozen-Bolzano) was approximately 480 mm (period 1989–2012) (Penna et al., 2021). Minimum average temperatures are below 0 ∘C during winter (from December to February), while maximum average temperatures can reach 24 ∘C in July (Penna et al., 2021). The soil in both orchards had a silty loam texture.
The sampling campaign was performed on 8 June 2017 during a period of water deficit. All samples were equally taken both at the right and the left field. Due to logistic issues and to collect samples when the transpiration fluxes were close to their minimum, samplings were carried out after sunset. Samples for water extraction by SPC and CVD were taken from the same position in the trees. More details can be found in Penna et al. (2021).
2.3 Ressi
Samples from beech (Fagus sylvatica) and chestnut (Castanea sativa) trees were collected in the 2.4 ha Ressi catchment in the Italian pre-Alps (Fig. 1) (Zuecco et al., 2014, 2021). The catchment is located at the foothills of the eastern Italian Alps (elevation range: 598–721 m a.s.l.) and is densely vegetated. The climate is humid temperate and the average annual precipitation (period 1992–2007) recorded by a weather station approximately 4.5 km from Ressi is 1695 mm yr−1 (Penna et al., 2015). Monthly distribution of rainfall is bimodal with peaks in spring and fall. The mean annual temperature is 9.7 ∘C; on average, the minimum monthly temperature is in January (1.2 ∘C) and the maximum in July (18.7 ∘C). The top 10 cm of the soil has a sandy clay loam texture; deeper in the profile, the soil has a sandy clay texture (Penna et al., 2015; Zuecco et al., 2021).
The sampling campaign was carried out on 5 July 2017 during a period of prolonged water deficit. Samples for plant water extraction were retrieved at sunrise from five beech and five chestnut trees at two sites in the lower part of the Ressi catchment. Samples for water extraction by SPC and CVD were taken from the same position in the trees. The sampling design aimed to replicate the sample collection in all the selected trees with the two investigated methodologies (Table 1). However, plant water extraction was not always possible by the SPC method because, in some cases (1 out of 5 chestnut samples and 2 out of 5 beech samples), the extracted plant water volume was not always enough for isotopic analysis. In addition, we discarded some plant water samples extracted by CVD, affected by injection issues during the isotopic analysis and for which we could not perform a second run of isotopic analyses due to the small water volume. Therefore, in this study, we reported only the isotopic data relative to the plant water extracted by both methods (i.e., SPC and CVD) from the same trees (Table 1).
3.1 Extraction of plant water: the SPC method
The SPC is an instrument normally used by tree physiologists to measure water potential in plant tissues (e.g., Scholander, 1966; Meiri et al., 1975; Donovan et al., 2003; Grossiord et al., 2017). Typically, SPC is used to determine plant water potential (Cochard et al., 2001) and, being a proxy of the tissue water content, it can signal the occurrence of water deficit. The basic working principle is the use of an external pressure to retrieve the water within the xylem conduits (Scholander et al., 1965; Turner, 1981; Castro Neto et al., 2004) (Fig. 2). In this study, we used the SPC to force water out of the twigs and collect water samples for isotopic analyses. The sampling material consisted of lignified twigs with a diameter ranging between 3 and 6 mm. Following previous studies (Penna et al., 2013, 2021), we kept the bark and the leaves attached to the twig. However, our technique does not represent the standard procedure for the extraction of xylem water which instead requires the removal of the bark and the phloem tissue (Geißler et al., 2019; Magh et al., 2020).
The setup for the plant water extraction consisted of a lignified twig with one or more leaves sealed inside the chamber, whereas the cut end of the twig was exposed to the atmosphere (Fig. 2). After connecting the SPC to the gas tank, a three-way control valve was turned to pressure and the metering valve was slowly opened to begin to pressurize the unit. A pressure equal to the water potential was applied until water flowed out of the cut end of the twig. For the SPC plant water extractions, we used a Pump-Up Chamber with a 2.0 MPa gauge (PMS Instrument Company, Oregon, USA) in Ahr/Aurino and Laas/Lasa and a SAPS II portable plant water status console (model 3115) with a 4.0 MPa gauge (Soilmoisture Equipment Corp., California, USA) in Ressi. The plant water was collected in 2 mL glass vials (which were immediately capped) by using pipettes or with the help of gravity (SPC was put on its side).
To extract water from the plant tissues, we had to apply a pressure of about 0.5 MPa in Laas/Lasa, 1.0–1.5 MPa in Ahr/Aurino, and 3.0 MPa in Ressi; the different plant water potentials indicate that the sampled vegetation in Ressi suffered higher water deficit conditions than the sampled plants in Ahr/Aurino and Laas/Lasa. The lower water deficit in Laas/Lasa than in the other two sites can be explained by the irrigation of the apple orchards during dry periods. The water extraction by the SPC method ended when we collected all the water flowing out from the twigs. The duration of the extraction was different among the samples (due to the different water deficit conditions), but we kept it as short as possible (less than 10 min) to minimize the evaporation. Note that the sampled volume was smaller than 200 µL during the sampling campaigns carried out for this study (Table 1). All the samples were stored in a fridge at 4 ∘C until the isotopic analyses.
3.2 Extraction of plant water: the CVD method
To extract plant water by CVD, we collected samples from different plant tissues along a branch in 12 mL glass Exetainer® vials (Labco Ltd., UK). After cutting the twigs from the trees, we removed all the leaves and other green tissues close to the leaves. Some of these leaves were collected in the vials for the extraction by CVD (i.e., CVD-L samples). CVD-L samples were used to determine the isotopic composition of the leaf water. The twig samples were lignified and approximately 85 mm long and 3–6 mm thick. For some of the twig samples, we kept the bark (i.e., twig with bark samples, abbreviated to CVD-TwB), whereas for others, bark was peeled using a knife (i.e., twig without bark samples, abbreviated to CVD-T; Table 1). CVD-TwB was used as the reference sample for the comparison with the SPC method. CVD-T represented plant water deprived of the phloem tissue. In the Ahr/Aurino and Laas/Lasa study sites, the diameters at the breast height of the alder and apple trees allowed us to collect wood core samples (abbreviated to CVD-WC), retrieved by an increment borer (phloem tissue was removed and the heartwood was not collected during the samplings). In Ressi, instead of wood cores (the sampling was not possible because of the small tree diameters and the location in a private land), we collected additional twig samples that were located close to the trunk (abbreviated to CVD-TcT). For these samples, we removed the bark with a knife. CVD-TcT samples were supposed to have older tissues and more dead cells than the twigs collected closer to the leaves (i.e., CVD-T and CVD-TwB).
The plant water volume of CVD samples was larger than the volume of SPC samples (Table 1), with a minimum of 100 µL (three CVD-WC samples from alder trees) and a maximum of 2690 µL (a CVD-L sample from an apple tree). All the samples for CVD were stored in a fridge at 4 ∘C until the extraction and the consequent isotopic analyses.
The CVD was performed in the laboratory of the Faculty of Science and Technology of the Free University of Bozen-Bolzano (Italy) (Fig. 3). The CVD system was developed based on the method of Koeniger et al. (2011). The system consisted of independent extraction–collection units where the capped sample vial was connected to a second empty vial (hereafter, collection vial) using a 1.56 mm diameter stainless steel capillary tube. After the preparation of the extraction–collection unit, the samples were frozen by immersing the sample vials in liquid nitrogen (approximately at –196 ∘C) to prevent loss of water vapor during evacuation (vials were evacuated to a pressure of 0.95 kPa). The sample vials were then loaded in an aluminum block (with slots for 10 vials) and heated to a temperature of 200 ∘C (Fig. 3). At the same time, during the extraction process, the bottom of the collection vials was immersed into the liquid nitrogen trap, which allowed for the evacuation of the sample from the heated vial and its condensation in the collection vial. All the individual plant samples were extracted at a temperature of 200 ∘C for an extraction time of 15 min per sample (Amin et al., 2021). A heat gun (at 300 ∘C) was used at the end of each extraction round to remove from the steel tube any water vapor trapped in the capillary tube. After the water had been quantitatively transferred from the plant tissue to the collection vial, vials were removed from the liquid nitrogen cold trap, defrosted at room temperature under perfect sealed conditions and stored in a refrigerator after labeling, and tightly wrapped with Parafilm® until the isotopic analysis. The exhausted vials were successively recovered in 100 ∘C oven for 24 h, while the capillary tubes were cleaned with acetone and then dried. All the plant samples were weighted before and after water extraction and after the oven-drying at 100 ∘C for 24 h to determine the extraction efficiency. We obtained an average extraction efficiency of 98.6 % (n=65), whereas the median was 100 %.
3.3 Isotopic analysis
Isotopic analyses were performed by isotope-ratio mass spectrometry (IRMS) at the Faculty of Science and Technology of the Free University of Bozen-Bolzano. All water samples were analyzed using an IRMS (Delta V Advantage Conflo IV, Thermo Fisher Scientific Inc., Waltham, Massachusetts, USA), coupled with a Thermo Scientific Gas Bench II to determine δ18O.
For δ18O, water samples were placed in Exetainer® vials and the headspace flushed by a 0.3 % CO2-He gas mixture of known isotopic composition. After an equilibration phase of 24 h, the headspace vapor phase was injected 8 times. δ2H was determined by direct injection of the sample into the IRMS through Thermo Scientific High Temperature Conversion Elemental Analyzer (TC/EA, Thermo Fisher Scientific Inc., Waltham, Massachusetts, USA), equipped with an autosampler (Thermo Scientific AI/AS 3000).
The samples were calibrated with standards relative to the Vienna Standard Mean Ocean Water. The standard deviation of the isotopic measurements performed by the IRMS was 2.5 ‰ and 0.10 ‰ for δ2H and δ18O, respectively.
3.4 Data analysis
The samples were grouped based on the extraction method (i.e., SPC and CVD) and plant species (i.e., alder, apple, chestnut, and beech trees). In addition, samples extracted by CVD were grouped based on the collected plant tissue (i.e., leaves (CVD-L), twig with bark (CVD-TwB), twig without bark (CVD-T), twig close to the trunk of the tree (CVD-TcT), and wood core (CVD-WC)). In total, we considered 24 groups of samples for data analyses. Although the reference comparison was between SPC and CVD-TwB samples, we applied the data analyses to all groups of samples to show whether, for this specific case study, there were marked isotopic differences among the waters extracted from the various plant tissues.
Data from the samples were plotted in the dual-isotope space, together with the Local Meteoric Water Lines (LMWLs) of the three study areas, obtained by the ordinary least squares regression (Marchina et al., 2020) to identify potential evaporated samples. For each sample, we computed the line-conditioned excess (lc-excess*), which considers the deviation from the LMWL and the uncertainty in the isotopic composition (Landwehr and Coplen, 2006), as follows:
where a and b are the slope and the intercept of the LMWLs for each of the three study sites (equations reported in Fig. 4), and S is the measurement uncertainty (Landwehr and Coplen, 2006). S was determined as follows:
where and are the typical standard deviations of the isotopic measurements (in our case, 2.5 ‰ and 0.10 ‰ for δ2H and δ18O, respectively). S resulted in 2.60, 2.61, and 2.63 in Ahr/Aurino, Laas/Lasa, and Ressi, respectively. lc-excess* values were used to investigate whether there was a marked offset of the samples from the LMWLs. Negative values of lc-excess* mean that the samples experienced isotopic fractionation by evaporation or other fractionation processes (these samples plot below the LMWL).
Scatterplots between SPC with CVD-T, CVD-TwB, CVD-TcT, and CVD-WC samples were used to assess differences (overestimation or underestimation) in the isotopic values. The Friedman repeated measures analysis of variance on ranks, paired with a multiple comparison test based on the Tukey method, was used to identify significant differences (at the 0.05 level) in the isotopic composition, lc-excess*, and volume of plant water extracted by the two methods and for the various tissues collected from alder and apple trees (these tests were not applied to chestnut and beech isotopic data because the paired samples were <4). The Welch two-sample t-test was used to assess whether the differences in the isotopic composition of SPC and CVD-L samples from alder and apple trees were significant (at the 0.05 level).
To evaluate differences in the isotopic composition between SPC and CVD samples while accounting for the uncertainty in the isotopic measurements, we computed the Z scores for each paired sample and isotope (Wassenaar et al., 2012; Orlowski et al., 2016b), as follows:
where CVD is the δ2H or δ18O value of the cryogenic extracted samples, SPC is the δ2H or δ18O value of the SPC samples, and SD is the typical standard deviation of the isotopic measurements. For the CVD samples, we distinguished the various plant tissues, i.e. CVD-T, CVD-TwB, CVD-TcT, and CVD-WC. Similar to Orlowski et al. (2016b), for Z score <2, we considered the difference between the extraction methods acceptable (i.e., the observed difference can be considered equal or lower than the uncertainty in the isotopic measurements), for 2<Z score <5, the difference was considered questionable, whereas for Z score >5, the difference was defined as unacceptable.
Scatterplots, the Friedman repeated measures analysis of variance on ranks (Scheff, 2016), and the Z score analysis were applied only to those groups of samples that were not greatly affected by evaporation (i.e., CVD-L samples were not considered). We applied the Friedman repeated measures analysis of variance on ranks instead of analysis of variance because the repeated samples were few and non-normally distributed. The statistical analyses and the plots were prepared using SigmaPlot, Microsoft Excel, and R.
Table 1Sample size, median sample volume, median isotopic composition, and median lc-excess* of the samples extracted by Scholander-type pressure chamber (SPC) and cryogenic vacuum distillation (CVD) from different plant tissues (L: leaves; T: twig without bark; TwB: twig with bark; WC: wood core; TcT: twig close to the trunk) and species in the three study sites (Ahr/Aurino, Laas/Lasa and Ressi). Note that SPC samples consisted of lignified twigs with bark and leaves attached to the twig, and the reference comparison is between SPC and CVD-TwB.
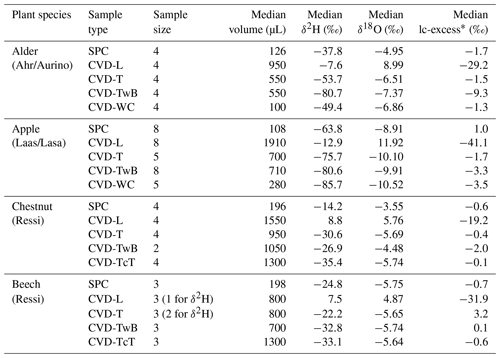
4.1 Isotopic variability across extraction methods and plant tissues
The volume of plant water extracted by the two methods and used for the isotopic analysis was significantly different (p<0.001, Friedman repeated measures analysis of variance on ranks). Specifically, the volume of CVD-L samples was larger compared to the volume of SPC samples (Table 1) and SPC samples had a significantly smaller volume compared to CVD-L, CVD-T, and CVD-TwB samples (p<0.05, pairs =16, Tukey test run without accounting for species differentiation).
The isotopic composition of plant water varied considerably across the different plant tissues (Table 1 and Fig. 4). We found that CVD-L samples were more enriched in heavy isotopes than all the other plant tissues samples and they plotted to the right side of the three LMWLs, highlighting a distinct evaporation signature (Fig. 4). Plant water extracted by SPC, CVD-T, CVD-TwB, CVD-WC, and CVD-TcT generally plotted close to the LMWLs, except for three CVD-TwB samples from alder trees that were more depleted in heavy isotopes and plotted on the right side of the LMWL and two samples from beech trees (one CVD-T and one CVD-TcT) that slightly plotted on the left side of the LMWL (Fig. 4). SPC samples were more enriched in heavy isotopes than CVD-T, CVD-TwB, and CVD-WC samples collected in Ahr/Aurino and Laas/Lasa, whereas differences between SPC and CVD samples (except for CVD-L) were less marked in Ressi for both beech and chestnut trees (Fig. 4). The Welch two-sample t-test, applied only to alder and apple tree samples, showed that there was a significant difference in δ2H and δ18O of SPC and CVD-L samples (p<0.001 for all four tests).
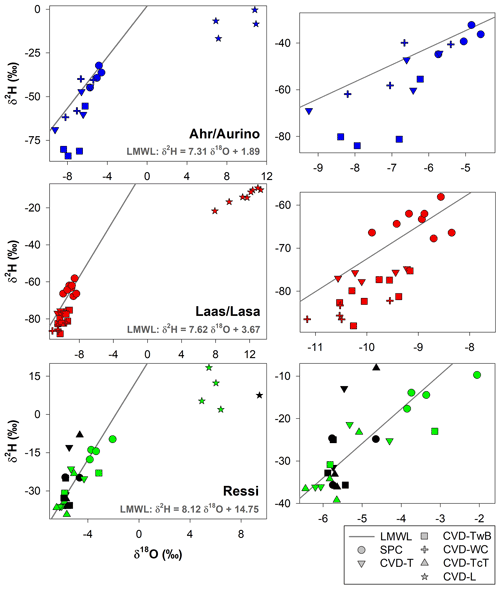
Figure 4Dual-isotope plot for plant water samples extracted by Scholander-type pressure chamber (SPC) and cryogenic vacuum distillation (CVD) for different plant tissues (CVD-T, CVD-TwB, CVD-WC, CVD-TcT, and CVD-L indicate samples extracted by CVD from twig without bark, twig with bark, wood core, twig close to the trunk of the tree, and leaves, respectively) and species (alder, apple, chestnut, and beech indicated in blue, red, green, and black, respectively). Local Meteoric Water Lines (LMWLs) of the three study sites are plotted in gray: Ahr/Aurino: (Engel et al., 2022), Laas/Lasa: (Penna et al., 2021), Ressi: (Marchina et al., 2020). The three plots on the right column represent a zoom in on SPC, CVD-T, CVD-TwB, CVD-WC, and CVD-TcT samples.
The relation between δ2H and δ18O of plant water extracted by SPC and CVD showed differences among plant tissues and the four species (Figs. 5 and 6). Indeed, we observed that most of the samples did not plot on the 1:1 line and there were very large differences between δ2H of SPC and CVD-TwB – the reference comparison – particularly for alder tree samples (the absolute differences varied between 16.1 ‰ and 48.9 ‰) and apple tree samples (the absolute differences varied between 12.0 ‰ and 21.7 ‰) (Fig. 5b). For alder, apple, and chestnut tree samples, we found that δ2H of SPC was always more positive than δ2H of CVD samples, except for CVD-L (Fig. 4). The δ2H of plant water collected from beech trees by CVD-T, CVD-TwB, and CVD-TcT was not systematically more enriched or depleted than δ2H of SPC samples.
Likewise, we found differences in δ18O values between SPC and CVD samples (Fig. 6). However, compared to δ2H, more samples plotted closer to the 1:1 line. The differences between SPC with CVD-T and CVD-TwB of beech samples were small (the median of the absolute differences was 0.22 ‰, n=6) and the samples plotted very close to the 1:1 line (Fig. 6a, b). SPC samples from alder, apple, and chestnut trees were less negative in δ18O than CVD samples, but for apple tree samples, the differences between SPC and CVD-TwB were relatively small (the median of the absolute differences was 0.57 ‰, n=8).
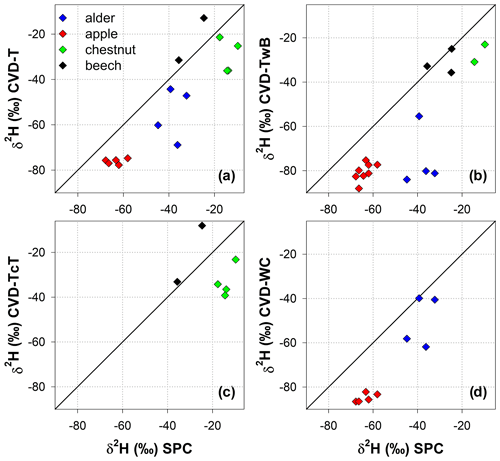
Figure 5Relation between δ2H values in plant water extracted by SPC (i.e., Scholander-type pressure chamber) and CVD (i.e., cryogenic vacuum distillation), grouped by plant tissue and species. The solid black lines represent the 1:1 line.
4.2 Effect of the extraction method on plant water isotopic composition
The Friedman repeated measures analysis of variance on ranks (applied to alder and apple isotopic data only) showed that there was a significant effect (with α=0.05) of the extraction method and plant tissue on δ2H and δ18O of plant water (Fig. 7). For alder trees, we found that SPC samples were significantly different in δ2H and δ18O from CVD-TwB samples (p<0.05, pairs =4, Tukey test). For apple trees, SPC samples differed in δ2H and δ18O from CVD-WC samples (p<0.05, pairs =5, Tukey test).
We observed that there was not a significant effect of the extraction method on lc-excess* (p>0.05, Friedman repeated measures analysis of variance on ranks) except for CVD-L samples (median lc-excess* was always very negative; Table 1). Besides CVD-L samples, a marked negative lc-excess* (larger than the uncertainty in the isotopic measurements) was found only for CVD-TwB samples collected from alder trees. Median lc-excess* was close to zero for chestnut and beech tree samples (CVD-T of beech samples even had positive values) and for some alder (SPC, CVD-T, and CVD-WC) and apple (SPC and CVD-T) tree samples (Fig. 7 and Table 1). Interestingly, SPC samples from apple trees had a positive median lc-excess* compared to the negative lc-excess* and larger offset from the LMWL of CVD-TwB and CVD-WC samples collected from the same plants (Fig. 7 and Table 1).
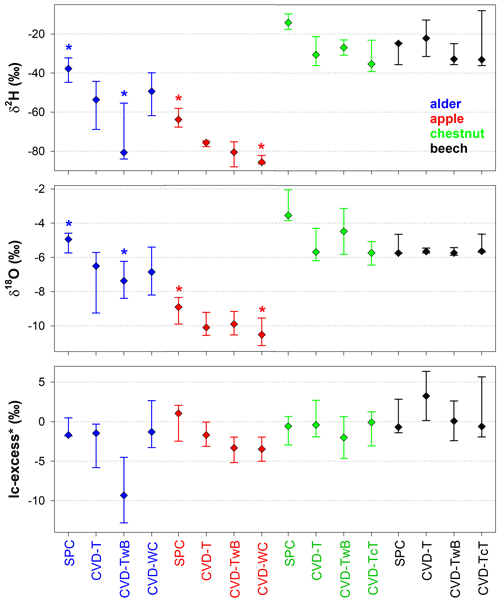
Figure 7Median isotopic composition and lc-excess* of plant water extracted by SPC (i.e., Scholander-type pressure chamber) and CVD (i.e., cryogenic vacuum distillation), grouped by plant tissue and species. Error bars represent the minimum and the maximum values. Asterisks above the dots indicate significantly different groups (p<0.05, multiple comparison test based on Tukey method run after the Friedman repeated measures analysis of variance on ranks).
4.3 Are the differences between SPC and CVD larger than the uncertainty in the isotopic measurements?
The Z score analysis showed that the differences between δ2H and δ18O of SPC and CVD samples were generally larger than the uncertainty in the isotopic measurements (Fig. 8). Due to the larger uncertainty in δ2H measurements compared to δ18O (based on the IRMS used in this study), we observed that the computed Z scores were smaller for δ2H than for δ18O.
For δ2H, Z scores varied between 0.1 (computed between SPC and CVD-TwB for samples collected from a beech tree in Ressi) and 19.6 (computed between SPC and CVD-TwB for samples collected from an alder tree in Ahr/Aurino). The median Z scores for δ2H were 6.0, 6.5, 6.6, and 7.6 computed between SPC with CVD-T, CVD-TwB, CVD-TcT, and CVD-WC, respectively; these median values indicate that more than 50 % of the Z scores were above the limit for questionable differences (Z score =5) between the extraction methods (Fig. 8a). For δ2H, only few Z scores (about 10 %) were lower than the upper limit for acceptable differences (Z score =2) between the methods. Overall, the smallest differences in δ2H (and Z scores) were found between SPC and CVD-T, followed by SPC and CVD-TwB (Fig. 8a).
For δ18O, Z scores varied between 0.1 (computed between SPC and CVD-T for samples collected from a beech tree in Ressi) and 46.6 (computed between SPC and CVD-T for samples collected from an alder tree in Ahr/Aurino). The median Z scores for δ18O were 12.4, 10.8, 19.8, and 16.1 computed between SPC with CVD-T, CVD-TwB, CVD-TcT, and CVD-WC, respectively; these results indicate that about 75 % of the Z scores were above the limit for questionable differences between the extraction methods (Fig. 8b). For δ18O, only few Z scores (less than 10 %) were lower than the upper limit for acceptable differences between the methods. The smallest differences in δ18O (and Z scores) were observed between SPC and CVD-TwB, followed by SPC and CVD-T (Fig. 8b).
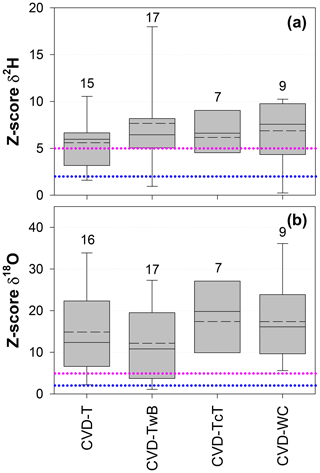
Figure 8Dimensionless Z score values for δ2H (a) and δ18O (b) grouped by sample types (CVD: cryogenic vacuum distillation; T: twig without bark; TwB: twig with bark; TcT: twig close to the trunk; WC: wood core). Samples from the four species were grouped together and numbers above the boxes represent the sample size. The boxes indicate the 25th and 75th percentile, the whiskers indicate the 10th and 90th percentile, whereas the horizontal solid and dashed lines within the box mark the median and the mean, respectively. The dotted blue and pink lines represent the upper limits for acceptable (Z score = 2) and questionable (Z score =5) differences, respectively, between the SPC (i.e., Scholander-type pressure chamber) and the CVD-extracted samples.
5.1 Advantages and limitations of water extraction by SPC
The SPC has the advantage of extracting plant water likely used for transpiration (Meiri et al., 1975; Grossiord et al., 2017). The water extraction by SPC can be applied directly in the field or in a laboratory after a proper handling and transport of the vegetation material in sealed bags. The procedure for the extraction of plant water is also simple because it does not require specific laboratory work (such as handling liquid nitrogen and transferring samples to different vials) in contrast to the CVD system. In addition, water extraction by SPC generally lasts a few minutes depending on the plant water potential, whereas the extraction by CVD could last from a few minutes (15 min in this study) up to hours (Millar et al., 2018). The easy and fast application (without extensive laboratory work) of the SPC for plant water extraction can be considered comparable to the simple and low-cost methods developed by Fischer et al. (2019).
Despite the advantages listed above, our sampling approach showed that water extraction by SPC is not always satisfactory in terms of sampling volume and extraction times. For instance, for some twig samples collected in Ressi during a dry period in July 2017, we had to apply a 3.0 MPa pressure for the extraction of at least 60 µL for the isotopic analysis by IRMS, and the whole sample extraction lasted about 10 min. The sampling procedure was also complicated by the extraction of few small water droplets and air bubbles. Conversely, the plant water extraction by CVD was performed for all the samples, generally obtaining sampling volumes much larger than 100 µL. Furthermore, plant water extracts obtained by SPC usually were darker (yellowish or even brownish) compared to water extracts by CVD. The dark color of the SPC plant water extracts suggests a possibly large concentration in organic compounds (Millar et al., 2018), likely due to a partial destruction of plant cell walls and/or a potential contamination by the phloem (we did not peel this tissue from the SPC samples). In our case, the sampling volume was not enough to quantify the concentration of organic compounds. Compared to this study, Geißler et al. (2019) performed a post-processing analysis by a spectral contamination identifier software to quantify the spectral contamination of organic compounds, and found that for six stem water samples from Acacia mellifera, the relative degree of interference from contaminants in the extracted water was clearly higher for CVD than SPC (Fig. S1 in Geißler et al., 2019).
5.2 Difference between the two techniques and implications for ecohydrological studies
Our results showed that twig samples (with leaves) obtained by SPC differently from the CVD-L samples did not show any offset compared to the LMWL of each site (Fig. 4). This might suggest that we did not extract significant volumes of leaf water which is typically subject to water–vapor exchanges with the low atmosphere (e.g., Cernusak et al., 2016; Benettin et al., 2021). The lc-excess* values of plant water extracted by SPC and CVD were generally close to zero (except for CVD-L and CVD-TwB samples collected from alder trees, Fig. 7), indicating a limited deviation from the LMWLs (generally smaller than the uncertainty in the isotopic measurements; Table 1 and Fig. 7), with even positive values for plant water samples extracted from some beech trees in Ressi. This observation indicates that either the applied methods did not alter the isotopic composition of plant water during the extraction (and did not determine a marked deviation from the LMWLs) or that both methods did not access plant water with a significant offset from the LMWLs. Despite the similarity in lc-excess* between samples extracted with the two methods (no significant differences were found by the p>0.05, Fig. 7), we observed that the plant water collected by the CVD method from alder, apple, and chestnut trees was always more depleted in heavy isotopes (both δ2H and δ18O) and in some cases, significantly different from plant water samples extracted by SPC (Figs. 5, 6 and 7). Our results are partly in contrast with the findings of Barbeta et al. (2022) who found a marked depletion in δ2H of plant water extracted by CVD compared to xylem water obtained by the cavitron technique, whereas we observed an offset for both isotopes (except for the comparison between SPC and CVD-TwB in beech tree samples, where the offset was very marked only for δ2H). As expected, given the different plant water domain accessed by the two methods, the water extracted by SPC and CVD showed differences in the isotopic composition among plant tissues, larger than the uncertainty in the isotopic measurements, and such differences were considered unacceptable in terms of Z scores (Fig. 8). As expected, these results are in contrast with those found by Geißler et al. (2019), who reported a large variability in δ18O but no statistical differences for six stem water samples of Acacia mellifera extracted by CVD and SPC. However, we must consider that Geißler et al. (2019) compared a limited number of samples and a different species and used samples deprived of the phloem tissue.
In our study, we attribute the observed isotopic differences between SPC and CVD samples to various factors, such as the possible effect of organic compounds on the isotopic composition of plant water (but we did not quantitatively verify this possible factor) and/or the plant water domain accessed by each method (e.g., more mobile plant water extracted by SPC vs. all plant water extracted by CVD). Millar et al. (2018) reported that different methods can extract different water domains within the plant, and CVD extracts up to 99 % of the water in a sample, i.e., CVD accesses total plant water. Conversely, SPC mainly extracts water present in the xylem conduits and given the much smaller sample volumes we collected by SPC than by CVD (on average ≈135 µL for SPC vs. ≈955 µL for CVD, Table 1), we likely accessed different plant water domains when using the two methods, with SPC pulling out more easily mobile water (i.e., xylem and inter-cellular water) than water stored in living cells. Even for CVD-TcT samples which were supposed to have older tissues and more dead cells than CVD-T and CVD-TwB, we need to consider that CVD could still extract significant water amounts stored in living xylem parenchyma cells, and the total ray and axial parenchyma tissue fractions can be 21.1±7.9 % (average ± standard deviation) in temperate angiosperm trees (Morris et al., 2016). Water uptaken by roots and transported in the xylem conduits can reach the leaves very rapidly and be available for transpiration, whereas the living cells (which are abundant in the leaves and other non-lignified tissues) may store water uptaken several days or even weeks before the sampling date (Sprenger et al., 2019). Therefore, water uptaken by roots in different periods and stored in different tissues might have very different isotopic compositions, a possible effect that both methods cannot clearly distinguish.
The ability of SPC and CVD to extract different plant water domains has implications for studies investigating the water sources exploited by plants for transpiration (e.g., Zhao et al., 2016; Barbeta et al., 2019; Allen and Kirchner, 2022; Barbeta et al., 2022). Indeed, significantly different isotopic compositions in the extracted plant water, which can also be related to the δ2H bias observed in the CVD extraction in recent studies by Chen et al. (2020) and Barbeta et al. (2022), can complicate the identification of the water sources contributing to transpiration and can result even in substantially different estimations of the contributing water sources (Barbeta et al., 2019; Allen and Kirchner, 2022). In this view, such ecohydrological studies should rely more on methods extracting apoplastic water representative of transpiration (like SPC) than on methods extracting, in addition to xylem water, also other plant water fractions (stored in living cells) that are likely much older (even decades, as reported in Zhang et al., 2017) than the actual xylem water. In addition, possible exchanges during transportation among different plant water domains would result in mixing of water having different ages and likely different isotopic composition (Ellsworth and Williams, 2007; Zhao et al., 2016).
Furthermore, our results show that the differences in the isotopic composition between SPC and CVD vary not only based on the plant tissue used for CVD, but also based on the plant species (Figs. 5, 6 and 7). Given the isotopic differences among various species and the results obtained by Geißler et al. (2019), more research is needed to compare multiple extraction methods (SPC should not only be compared to CVD, but as well as to direct vapor equilibration, microwave extraction, centrifugation, cavitron flow-rotor etc., that might access different plant water domains). Future inter-method comparisons should be carried out across various environments and plant species to investigate factors able to alter the isotopic composition of plant water during the extraction and/or potentially different plant water domains accessed by each method in order to elaborate standard protocols for ecohydrological research relying on the isotopic signature of plant water.
5.3 Limitations of this study
Our results contribute to the pressing need of comparing different plant water extraction techniques to understand which plant water domains are accessed by different methods (Penna et al., 2018). Despite the importance of our findings for the isotope ecohydrological community, we acknowledge some limitations in the experimental setup which may impact the interpretation of the results.
Firstly, our experiment was not designed to test whether plant water extracted by SPC from twigs with or without bark had a significantly different isotopic composition. Contrary to Geißler et al. (2019) who performed their experiment after we performed ours, we did not remove bark and the leaves attached to the twig. However, we found no direct influence of leaf water isotopic composition on our SPC samples (no deviation from LMWLs, see Fig. 4), and therefore, we could compare plant water extracted by SPC to plant water obtained by CVD-TwB. Nonetheless, our results are not directly comparable to the findings by Geißler et al. (2019), and future research should aim to test whether SPC is able to extract phloem from twigs with bark and green tissues and whether there is a significant isotopic difference with SPC samples obtained from twigs without bark.
Secondly, our experimental design did not include the quantification of organic compounds and the water volume obtained for SPC samples was not enough to carry out such analyses. The quantification of organic compounds in SPC samples might be a useful indicator of possible destruction of cell walls. At the same time, the analysis of organic compounds might give insight on the possible alteration of the original isotopic composition of plant water. Because we were not able to quantify the concentration of organic compounds in our samples, we recommend that future inter-method studies should compare the isotopic composition of plant water extracted by SPC and CVD and the measured concentration of organic compounds.
Another limitation of our experimental setup is represented by the sampling time which differed due to logistic constraints at the three study sites. Indeed, we cannot exclude a variability in the daily dehydration–rehydration cycles inside the stems related to the phloem–xylem water transfer (Pfautsch et al. , 2015) during the two sampling times considered in this study (i.e., after sunset and at sunrise). A different phase in the phloem–xylem water transfer might have determined a different contamination of phloem during the SPC extraction at the three sites. Therefore, future inter-method studies should try to minimize the difference in sampling times for the collection of the various samples or design experiments should consider multiple sampling campaigns during the day and the daily variation in the phloem–xylem water transfer as a factor potentially affecting the isotopic composition of the plant water.
Finally, based on our experimental setup, we were not able to determine exactly which plant water domain was accessed by SPC. SPC samples were more comparable to CVD-TwB samples because we did not remove bark and our results confirmed that there was no influence of evaporated leaf water on the SPC samples. Therefore, similar to CVD-TwB, we cannot exclude that our SPC samples were contaminated by the extraction of water from the phloem tissue. However, the smaller sample volumes we collected by SPC compared to CVD (Table 1), and the different isotopic composition, particularly shown by the Z score analysis (Fig. 8), indicate that SPC tended to access more easily the mobile xylem water and inter-cellular water than the less mobile intra-cellular, cell wall, and organelle-constrained water (Millar et al., 2018). Despite this, our study did not resolve whether SPC was able to extract all the less mobile plant water (besides likely cell walls) and whether the results were affected by other factors, such as the presence of organic compounds or the sampling time. Given that we were not able to determine exactly the plant water domains accessed by SPC, future comparison studies between the SPC and CVD techniques should carefully consider the sample types (both with bark and without bark to assess whether SPC extracts phloem), the quantification of organic compounds, and the extraction of plant water using different external pressures. By applying different external pressures to plants not suffering from high water deficit and under the assumption that isotopic composition differs according to plant water mobility, it may be possible to test whether SPC extracts mobile plant water only when a low external pressure is applied whereas a mixture of water having variable mobility (and thus, age) is obtained during the application of higher pressures.
Our results indicate that the isotopic composition of plant water extracted by SPC and CVD can be significantly different. While SPC and most of the CVD samples (except for CVD applied to leaves) did not exhibit an evaporative signature, there was a large isotopic variability among the samples. We found that for beech tree samples, the difference in both δ2H and δ18O obtained by the two extraction methods was smaller compared to the difference observed for alder, apple, and chestnut tree samples. Specifically, the isotopic composition of alder, apple, and chestnut plant water extracted by SPC was more enriched in heavy isotopes compared to samples obtained by CVD applied to twigs or wood cores. Based on these results, we conclude that SPC accesses only the more mobile part of the plant water fraction that CVD does. Therefore, studies aiming to quantify the relative contribution of the water sources to transpiration should rely more on the isotopic composition of xylem water transpiring at the moment of the sampling or during the sampling day (which is theoretically sampled by SPC), than the isotopic composition of total plant water (sampled by CVD), which also contains a fraction of water that could be stored in plant tissues for a longer time. Based on our findings, we call for future research investigating the same methods across more plant species and quantifying the organic compounds in both SPC and CVD samples to determine the effect on the isotopic composition of plant water.
Data are available from the corresponding author upon reasonable request.
DP conceptualized the methodological comparison between the two methods. JF, GZ, and ME designed the research. JF and ME collected field data in the Ahr/Aurino and Laas/Lasa sites, whereas CM, GZ, and AA carried out the field campaigns in the Ressi catchment. JF and AA performed the plant water extraction by the cryogenic vacuum distillation. FS and DZ provided support for the sampling in Laas/Lasa and the laboratory activities. VC and TA provided technical support and comments for the plant water extraction by the Scholander-type pressure chamber. GZ analyzed the data set and prepared the first draft of the manuscript with contributions from AA, JF, ME, and CM. All the authors contributed to the editing of the manuscript. FC, MB, and MT funded the research.
The contact author has declared that none of the authors has any competing interests.
Publisher’s note: Copernicus Publications remains neutral with regard to jurisdictional claims in published maps and institutional affiliations.
This article is part of the special issue “Water, isotope and solute fluxes in the soil–plant–atmosphere interface: investigations from the canopy to the root zone”. It is a result of the EGU General Assembly 2018, Vienna, Austria, 8–13 April 2018.
The authors would like to thank Christian Ceccon for the isotopic analyses and the support during the laboratory activities at the Free University of Bozen-Bolzano (Italy).
This research has been supported by the Fondazione Cassa di Risparmio di Padova e Rovigo (Italy) (project “Ecohydrological Dynamics and Water Pathways in Forested Catchments”, Bando Starting Grants 2015), by the Italian MIUR project (PRIN 2017) “WATer mixing in the critical ZONe: observations and predictions under environmental changes-WATZON” (grant no. 2017SL7ABC), by the Free University of Bozen-Bolzano (project RIVERMOOD) and by the Department of Innovation, Research and University of the Autonomous Province of Bozen-Bolzano within the NOI Capacity Building II funding frame (Decision 864, 4 September 2018, project “Parco Tecnologico-Tecnologie Ambientali”).
This paper was edited by Josie Geris and reviewed by three anonymous referees.
Aguzzoni, A., Engel, M., Zanotelli, D., Penna, D., Comiti, F., and Tagliavini, M.: Water uptake dynamics in apple trees assessed by an isotope labeling approach, Agr. Water Manage., 266, 107572, https://doi.org/10.1016/j.agwat.2022.107572, 2022. a
Allen, S. T. and Kirchner, J. W.: Potential effects of cryogenic extraction biases on plant water source partitioning inferred from xylem-water isotope ratios, Hydrol. Process., 36, e14483, https://doi.org/10.1002/hyp.14483, 2022. a, b, c, d
Allen, S. T., Kirchner, J. W., Braun, S., Siegwolf, R. T. W., and Goldsmith, G. R.: Seasonal origins of soil water used by trees, Hydrol. Earth Syst. Sci., 23, 1199–1210, https://doi.org/10.5194/hess-23-1199-2019, 2019. a
Amin, A., Zuecco, G., Geris, J., Schwendenmann, L., McDonnell, J. J., Borga, M., and Penna, D.: Depth distribution of soil water sourced by plants at the global scale: a new direct inference approach, Ecohydrology, 13, e2177, https://doi.org/10.1002/eco.2177, 2020. a
Amin, A., Zuecco, G., Marchina, C., Engel, M., Penna, D., McDonnell, J. J., and Borga M.: No evidence of isotopic fractionation in olive trees (Olea europaea): a stable isotope tracing experiment, Hydrolog. Sci. J., 66, 2415–2430, https://doi.org/10.1080/02626667.2021.1987440, 2021. a
Andreoli, A., Chiaradia, E. A., Cislaghi, A., Bischetti, G. B., and Comiti, F.: Roots reinforcement by riparian trees in restored rivers, Geomorphology, 370, 107389, https://doi.org/10.1016/j.geomorph.2020.107389, 2020. a
Barbeta, A., Jones, S. P., Clavé, L., Wingate, L., Gimeno, T. E., Fréjaville, B., Wohl, S., and Ogée, J.: Unexplained hydrogen isotope offsets complicate the identification and quantification of tree water sources in a riparian forest, Hydrol. Earth Syst. Sci., 23, 2129–2146, https://doi.org/10.5194/hess-23-2129-2019, 2019. a, b, c
Barbeta, A., Gimeno, T. E., Clavé, L., Fréjaville, B., Jones, S. P., Delvigne, C., Wingate, L., and Ogée, J.: An explanation for the isotopic offset between soil and stem water in a temperate tree species, New Phytol., 227, 766–779, https://doi.org/10.1111/nph.16564, 2020. a
Barbeta, A., Burlett, R., Martín-Gómez, P., Fréjaville, B., Devert, N., Wingate, L., Domec, J.-C., and Ogée, J.: Evidence for distinct isotopic compositions of sap and tissue water in tree stems: consequences for plant water source identification, New Phytol., 233, 1121–1132, https://doi.org/10.1111/nph.17857, 2022. a, b, c, d, e, f, g, h
Benettin, P., Nehemy, M. F., Cernusak, L. A., Kahmen, A., and McDonnell, J. J.: On the use of leaf water to determine plant water source: A proof of concept, Hydrol. Process., 35, e14073, https://doi.org/10.1002/hyp.14073, 2021. a
Beyer, M. and Penna D.: On the spatio-temporal under-representation of isotopic data in ecohydrological studies, Front. Water, 3, 643013, https://doi.org/10.3389/frwa.2021.643013, 2021. a
Böttcher, G., Brumsack, H., Heinrichs, H., and Pohlmann, M.: A new high pressure squeezing technique for pore fluid extraction from terrestrial soils, Water Air Soil Pollut., 94, 289–296, https://doi.org/10.1007/BF02406064, 1997. a
Bowling, D. R., Schulze, E. S., and Hall, S.J.: Revisiting streamside trees that do not use stream water: can the two water worlds hypothesis and snowpack isotopic effects explain a missing water source?, Ecohydrology, 10, e1771, https://doi.org/10.1002/eco.1771, 2017. a
Brooks, R. J., Barnard, H. R., Coulombe, R., and McDonnell, J. J.: Ecohydrologic separation of water between trees and streams in a Mediterranean climate, Nat. Geosci., 3, 100–104, https://doi.org/10.1038/ngeo722, 2010. a
Campana, D., Marchese, E., Theule, J. I., and Comiti, F.: Channel degradation and restoration of an Alpine river and related morphological changes, Geomorphology, 221, 230–241, https://doi.org/10.1016/j.geomorph.2014.06.016, 2014. a
Castro Neto, M. T., Reinhardt, D. H., and da S. Ledo, C.A.: Determination of water potential on mango trees by pressure chamber, Acta Hortic., 645, 425–427, https://doi.org/10.17660/ActaHortic.2004.645.53, 2004. a
Cernusak, L. A., Barbour, M. M., Arndt, S. K., Cheesman, A. W., English, N. B., Feild, T. S., Helliker, B. R., Holloway-Phillips, M. M., Holtum, J. A. M., Kahmen, A., McInerney, F. A., Munksgaard, N. C., Simonin, K. A., Song, X., Stuart-Williams, H., West, J. B., and Farquhar, G. D.: Stable isotopes in leaf water of terrestrial plants, Plant Cell Environ., 39, 1087–1102, https://doi.org/10.1111/pce.12703, 2016. a
Chen, Y., Helliker, B. R., Tang, X., Li, F., Zhou, Y., and Song, X.: Stem water cryogenic extraction biases estimation in deuterium isotope composition of plant source water, P. Natl. Acad. Sci. USA, 117, 33345–33350, https://doi.org/10.1073/pnas.2014422117, 2020. a, b, c
Cochard, H., Forestier, S., and Améglio, T.: A new validation of the Scholander pressure chamber technique based on stem diameter variations, J. Exp. Bot., 52, 1361–1365, https://doi.org/10.1093/jxb/52.359.1361, 2001. a
Craig, H.: Isotopic variations in meteoric waters, Science, 133, 1702–1703, https://doi.org/10.1126/science.133.3465.1702, 1961. a
Dansgaard, W.: The abundance of O18 in atmospheric water and water vapour, Tellus, 5, 461–469, https://doi.org/10.1111/j.2153-3490.1953.tb01076.x, 1953. a
Donovan, L. A., Richards, J. H., and Linton, M. J.: Magnitude and mechanisms of disequilibrium between predawn plant and soil water potentials, Ecology, 84, 463–470, https://doi.org/10.1890/0012-9658(2003)084[0463:MAMODB]2.0.CO;2, 2003. a
Dubbert, M., Caldeira, M.C., Dubbert, D., and Werner, C.: A pool-weighted perspective on the two-water-worlds hypothesis, New Phytol., 222, 1271–1283, https://doi.org/10.1111/nph.15670, 2019. a
Ellsworth, P. Z. and Williams, D. G.: Hydrogen isotope fractionation during water uptake by woody xerophytes, Plant Soil, 291, 93–107, https://doi.org/10.1007/s11104-006-9177-1, 2007. a, b
Engel, M., Frentress, J., Penna, D., Andreoli, A., van Meerveld, I., Zerbe, S., Tagliavini, M., and Comiti, F.: How do geomorphic characteristics affect the source of tree water uptake in restored river floodplains?, Ecohydrology, 15, e2443, https://doi.org/10.1002/eco.2443, 2022. a, b
Fischer, B. M. C., Frentress, J., Manzoni, S., Cousins, S. A. O., Hugelius, G., Greger, M., Smittenberg, R. H., and Lyon, S. W.: Mojito, anyone? An exploration of low-tech plant water extraction methods for isotopic analysis using locally-sourced materials, Front. Earth Sci., 7, 150, https://doi.org/10.3389/feart.2019.00150, 2019. a, b, c, d, e
Geißler, K., Heblack, J., Uugulu, S., Wanke, H., and Blaum, N.: Partitioning of water between differently sized shrubs and potential groundwater recharge in a semiarid savanna in Namibia, Front. Plant Sci., 10, 1411, https://doi.org/10.3389/fpls.2019.01411, 2019. a, b, c, d, e, f, g, h, i, j, k
Grossiord, C., Sevanto, S., Adams, H. D., Collins, A. D., Dickman, L. T., McBranch, N., Michaletz, S. T., Stockton, E. A., Vigil, M., and McDowell, N. G.: Precipitation, not air temperature, drives functional responses of trees in semi‐arid ecosystems, J. Ecol., 105, 163–175, https://doi.org/10.1111/1365-2745.12662, 2017. a, b, c
Kerstel, E. R. T., van Trigt, R., Reuss, J., and Meijer, H. A. J.: Simultaneous determination of the 2H 1H, 17O 16O, and 18O 16O isotope abundance ratios in water by means of laser spectrometry, Anal. Chem., 71, 5297–5303, https://doi.org/10.1021/ac990621e, 1999. a
Klaus, J. and McDonnell, J. J.: Hydrograph separation using stable isotopes: Review and evaluation, J. Hydrol., 505, 47–64, https://doi.org/10.1016/j.jhydrol.2013.09.006, 2013. a
Koeniger, P., Marshall, J. D., Link, T., and Mulch, A.: An inexpensive, fast, and reliable method for vacuum extraction of soil and plant water for stable isotope analyses by mass spectrometry, Rapid Commun. Mass Spectrom., 25, 3041–3048, https://doi.org/10.1002/rcm.5198, 2011. a, b, c, d
Landwehr, J. M. and Coplen, T. B.: Line-conditioned excess: A new method for characterizing stable hydrogen and oxygen isotope ratios in hydrologic systems, in: International Conference on Isotopes in Environmental Studies, Aquatic Forum 2004, Monaco, 25-29 October 2004, IAEA Vienna, 132–135, https://www-pub.iaea.org/MTCD/publications/PDF/CSP_26_web.pdf (last access: 7 June 2022), 2006. a, b
Liu, J., Shen, L., Wang, Z., Duan, S., Wu, W., Peng, X., Wu, C., and Jiang, Y.: Response of plants water uptake patterns to tunnels excavation based on stable isotopes in a karst trough valley, J. Hydrol., 571, 485–493, https://doi.org/10.1016/j.jhydrol.2019.01.073, 2019a. a
Liu, Y., Zhang, X., Zhao, S., Ma, H., Qi, G., and Guo, S.: The depth of water taken up by walnut trees during different phenological stages in an irrigated arid hilly area in the Taihang Mountains, Forests, 10, 121, https://doi.org/10.3390/f10020121, 2019b. a
Magh, R.-K., Eiferle, C., Burzlaff, T., Dannenmann, M., Rennenberg, H., and Dubbert, M.: Competition for water rather than facilitation in mixed beech-fir forests after drying-wetting cycle, J. Hydrol., 587, 124944, https://doi.org/10.1016/j.jhydrol.2020.124944, 2020. a, b
Marchina, C., Zuecco, G., Chiogna, G., Bianchini, G., Carturan, L., Comiti, F., Engel, M., Natali, C., Borga, M., and Penna, D.: Alternative methods to determine the δ2H-δ18O relationship: an application to different water types, J. Hydrol., 587, 124951, https://doi.org/10.1016/j.jhydrol.2020.124951, 2020. a
Marshall, J. D., Cuntz, M., Beyer, M., Dubbert, M., and Kuehnhammer, K.: Borehole equilibration: Testing a new method to monitor the isotopic composition of tree xylem water in situ, Front. Plant Sci., 11, 358, https://doi.org/10.3389/fpls.2020.00358, 2020. a
McDonnell, J. J.: The two water worlds hypothesis: ecohydrological separation of water between streams and trees?, WIREs Water, 1, 323–329, https://doi.org/10.1002/wat2.1027, 2014. a
Meiri, A., Plaut, Z., and Shimshi, D.: The use of the pressure chamber technique for measurement of the water potential of transpiring plant organs, Physiol. Plantarum, 35, 72–76, https://doi.org/10.1111/j.1399-3054.1975.tb03870.x, 1975. a, b, c
Millar, C., Pratt, D., Schneider, D. J., and McDonnell, J.J.: A comparison of extraction systems for plant water stable isotope analysis, Rapid Commun. Mass Spectrom., 32, 1031–1044, https://doi.org/10.1002/rcm.8136, 2018. a, b, c, d, e, f, g, h, i, j
Morris, H., Plavcová, L., Cvecko, P., Fichtler, E., Gillingham, M. A. F., Martínez-Cabrera, H. I., McGlinn, D. J., Wheeler, E., Zheng, J., Ziemińska, K., and Jansen, S.: A global analysis of parenchyma tissue fractions in secondary xylem of seed plants, New Phytol., 209, 1553–1565, https://doi.org/10.1111/nph.13737, 2016. a
Munksgaard, N. C., Cheesman, A. W., Wurster, C. M., Cernusak, L. A., and Bird, M. I.: Microwave extraction-isotope ratio infrared spectroscopy (MEIRIS): a novel technique for rapid extraction and in-line analysis of δ18O and δ2H values of water in plants, soils and insects, Rapid Commun Mass Spectrom., 28, 2151–2161, https://doi.org/10.1002/rcm.7005, 2014. a
Oerter, E. J., Siebert, G., Bowling, D. R., and Bowen, G.: Soil water vapour isotopes identify missing water source for streamside trees, Ecohydrology, 12, e2083, https://doi.org/10.1002/eco.2083, 2019. a
Orlowski, N., Frede, H.-G., Brüggemann, N., and Breuer, L.: Validation and application of a cryogenic vacuum extraction system for soil and plant water extraction for isotope analysis, J. Sens. Sens. Syst., 2, 179–193, https://doi.org/10.5194/jsss-2-179-2013, 2013. a, b
Orlowski, N., Breuer, L., and McDonnell, J. J.: Ecohydrology Bearings – Invited Commentary Critical issues with cryogenic extraction of soil water for stable isotope analysis, Ecohydrology, 9, 3–10, https://doi.org/10.1002/eco.1722, 2016a. a
Orlowski, N., Pratt, D. L., and McDonnell, J. J.: Intercomparison of soil pore water extraction methods for stable isotope analysis, Hydrol. Process., 30, 3434–3449, https://doi.org/10.1002/hyp.10870, 2016b. a, b
Orlowski, N., Breuer, L., Angeli, N., Boeckx, P., Brumbt, C., Cook, C. S., Dubbert, M., Dyckmans, J., Gallagher, B., Gralher, B., Herbstritt, B., Hervé-Fernández, P., Hissler, C., Koeniger, P., Legout, A., Macdonald, C. J., Oyarzún, C., Redelstein, R., Seidler, C., Siegwolf, R., Stumpp, C., Thomsen, S., Weiler, M., Werner, C., and McDonnell, J. J.: Inter-laboratory comparison of cryogenic water extraction systems for stable isotope analysis of soil water, Hydrol. Earth Syst. Sci., 22, 3619–3637, https://doi.org/10.5194/hess-22-3619-2018, 2018. a, b
Penna, D., Stenni, B., Šanda, M., Wrede, S., Bogaard, T. A., Gobbi, A., Borga, M., Fischer, B. M. C., Bonazza, M., and Chárová, Z.: On the reproducibility and repeatability of laser absorption spectroscopy measurements for δ2H and δ18O isotopic analysis, Hydrol. Earth Syst. Sci., 14, 1551–1566, https://doi.org/10.5194/hess-14-1551-2010, 2010. a
Penna, D., Oliviero, O., Assendelft, R., Zuecco, G., van Meerveld, I., Anfodillo, T., Carraro, V., Borga, M., and Dalla Fontana, G.: Tracing the water sources of trees and streams: isotopic analysis in a small pre-alpine catchment, Procedia Environ. Sci., 19, 106–112, https://doi.org/10.1016/j.proenv.2013.06.012, 2013. a, b
Penna, D., van Meerveld, H. J., Oliviero, O., Zuecco, G., Assendelft, R. S., Dalla Fontana, G., and Borga, M.: Seasonal changes in runoff generation in a small forested mountain catchment, Hydrol. Process., 29, 2027–2042, https://doi.org/10.1002/hyp.10347, 2015. a, b
Penna, D., Hopp, L., Scandellari, F., Allen, S. T., Benettin, P., Beyer, M., Geris, J., Klaus, J., Marshall, J. D., Schwendenmann, L., Volkmann, T. H. M., von Freyberg, J., Amin, A., Ceperley, N., Engel, M., Frentress, J., Giambastiani, Y., McDonnell, J. J., Zuecco, G., Llorens, P., Siegwolf, R. T. W., Dawson, T. E., and Kirchner, J. W.: Ideas and perspectives: Tracing terrestrial ecosystem water fluxes using hydrogen and oxygen stable isotopes – challenges and opportunities from an interdisciplinary perspective, Biogeosciences, 15, 6399–6415, https://doi.org/10.5194/bg-15-6399-2018, 2018. a, b
Penna, D., Geris, J., Hopp, L., and Scandellari, F.: Water sources for root water uptake: Using stable isotopes of hydrogen and oxygen as a research tool in agricultural and agroforestry systems, Agr. Ecosyst. Environ., 291, 106790, https://doi.org/10.1016/j.agee.2019.106790, 2020. a
Penna, D., Zanotelli, D., Scandellari, F., Aguzzoni, A., Engel, M., Tagliavini, M., and Comiti, F.: Water uptake of apple trees in the Alps: Where does irrigation water go?, Ecohydrology, 14, e2306, https://doi.org/10.1002/eco.2306, 2021. a, b, c, d
Peters, L. I. and Yakir, D.: A direct and rapid leaf water extraction method for isotopic analysis, Rapid Commun. Mass Spectrom., 22, 2929–2936, https://doi.org/10.1002/rcm.3692, 2008. a
Pfautsch, S., Renard, J., Tjoelker, M. G., and Salih, A.: Phloem as capacitor: radial transfer of water into xylem of tree stems occurs via symplastic transport in ray parenchyma, Plant Physiol., 167, 963–971, https://doi.org/10.1104/pp.114.254581, 2015. a
Qiu, X., Zhang, M., Wang, S., Evaristo, J., Argiriou, A. A., Guo, R., Chen, R., Meng, H., Che, C., and Qu, D.: The test of the ecohydrological separation hypothesis in a dry zone of the northeastern Tibetan Plateau, Ecohydrology, 12, e2077, https://doi.org/10.1002/eco.2077, 2019. a
Quade, M., Klosterhalfen, A., Graf, A., Brüggemann, N., Hermes, N., Vereecken, H., and Rothfuss, Y.: In-situ monitoring of soil water isotopic composition for partitioning of evapotranspiration during one growing season of sugar beet (Beta vulgaris), Agr. Forest Meteorol., 266–267, 53–64, https://doi.org/10.1016/j.agrformet.2018.12.002, 2019. a
Scheff, S. W.: Fundamental statistical principles for the neurobiologist: A survival guide, Academic Press, https://doi.org/10.1016/C2015-0-02471-6, 2016. a
Scholander, P. F.: The role of solvent pressure in osmotic systems, P. Natl. Acad. Sci. USA, 55, 1407–1414, https://doi.org/10.1073/pnas.55.6.1407, 1966. a, b
Scholander, P. F., Bradstreet, E. D., Hemmingsen, E. A., and Hammel, H. T.: Sap pressure in vascular plants, Science, 148, 339–346, https://doi.org/10.1126/science.148.3668.339, 1965. a
Sprenger, M., Herbstritt, B., and Weiler, M.: Established methods and new opportunities for pore water stable isotope analysis, Hydrol. Process., 29, 5174–5192, https://doi.org/10.1002/hyp.10643, 2015. a
Sprenger, M., Stumpp, C., Weiler, M., Aeschbach, W., Allen, S. T., Benettin, P., Dubbert, M., Hartmann, A., Hrachowitz, M., Kirchner, J. W., McDonnell, J. J., Orlowski, N., Penna, D., Pfahl, S., Rinderer, M., Rodriguez, N., Schmidt, M., and Werner, C.: The demographics of water: A review of water ages in the critical zone, Rev. Geophys., 57, 800–834, https://doi.org/10.1029/2018RG000633, 2019. a, b, c
Turner, N. C.: Technique and experimental approaches for the measurement of plant water status, Plant Soil, 58, 339–366, https://doi.org/10.1007/BF02180062, 1981. a
Volkmann, T. H. M., Kühnhammer, K., Herbstritt, B., Gessler, A., and Weiler, M.: A method for in situ monitoring of the isotope composition of tree xylem water using laser spectroscopy, Plant Cell Environ., 39, 2055–2063, https://doi.org/10.1111/pce.12725, 2016. a
von Freyberg, J., Studer, B., and Kirchner, J. W.: A lab in the field: high-frequency analysis of water quality and stable isotopes in stream water and precipitation, Hydrol. Earth Syst. Sci., 21, 1721–1739, https://doi.org/10.5194/hess-21-1721-2017, 2017. a
Wassenaar, L. I., Ahmad, M., Aggarwal, P., van Duren, M., Pöltenstein, L., Araguas, L., and Kurttas, T.: Worldwide proficiency test for routine analysis of δ2H and δ18O in water by isotope-ratio mass spectrometry and laser absorption spectroscopy, Rapid Commun. Mass Spectrom., 26, 1641–1648, https://doi.org/10.1002/rcm.6270, 2012. a
Zhang, Z. Q., Evaristo, J., Li, Z., Si, B. C., and McDonnell, J. J.: Tritium analysis shows apple trees may be transpiring water several decades old, Hydrol. Process., 31, 1196–1201, https://doi.org/10.1002/hyp.11108, 2017. a
Zhang, Z., Yu, K., Siddique, K. H. M., and Nan, Z.: Phenology and sowing time affect water use in four warm-season annual grasses under a semi-arid environment, Agr. Forest Meteorol., 269–270, 257–269, https://doi.org/10.1016/j.agrformet.2019.02.027, 2019. a
Zhao, L., Wang, L., Cernusak, L. A., Liu, X., Xiao, H., Zhou, M., and Zhang, S.: Significant difference in hydrogen isotope composition between xylem and tissue water in Populus Euphratica, Plant Cell Environ., 39, 1848–1857, https://doi.org/10.1111/pce.12753, 2016. a, b, c, d
Zuecco, G., Penna, D., van Meerveld, H. J., Hopp, L., Dalla Fontana, G., and Borga, M.: Comparison of two different types of throughfall collectors, Die Bodenkultur, 65, 51–56, 2014. a
Zuecco, G., Marchina, C., Gelmini, Y., Amin, A., van Meerveld, H. J., Penna, D., and Borga, M.: Ressi experimental catchment: ecohydrological research in the Italian pre-Alps, Hydrol. Process., 35, e14095, https://doi.org/10.1002/hyp.14095, 2021. a, b