the Creative Commons Attribution 4.0 License.
the Creative Commons Attribution 4.0 License.
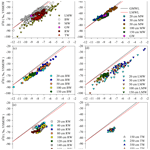
Insights into the isotopic mismatch between bulk soil water and Salix matsudana Koidz trunk water from root water stable isotope measurements
Ying Zhao
Li Wang
Increasing numbers of field studies have detected isotopic mismatches between plant trunk water and its potential sources. However, the cause of these isotopic offsets is not clear, and it is uncertain whether they occur during root water uptake or during water transmission from root to trunk. Thus, we measured the specific isotopic composition (δ2H and δ18O) of each component (e.g. bulk soil water, mobile water, groundwater, trunk water and root water of Salix matsudana Koidz trees) in the soil–root–trunk continuum with a resolution of about 3 days. We report three main findings. First, we detected a clear separation between the isotopic compositions of mobile water and bulk soil water, but the distinction between mobile water and bulk soil water gradually decreased with increasing soil depth. Second, root water composition deviated from bulk soil water isotopic composition but overlapped with the composition derived for less mobile water. The maximum differences in δ2H and δ18O between bulk soil water and root water were −8.6 ‰ and −1.8 ‰, respectively. Third, trunk water was only isotopically similar to root water at 100–160 cm depths, and it remained stable during the experimental period, suggesting that the trees consistently used the stable deep water source. In conclusion, the isotopic offset between bulk soil water and trunk water of S. matsudana reflected an isotopic mismatch between root water and bulk soil water associated with the heterogeneity of the soil water. Our results illuminate relationships between the isotopic compositions of soil waters of various mobilities, root water and trunk water that may be useful for advancing our understanding of root water uptake and transport.
- Article
(5724 KB) - Full-text XML
-
Supplement
(495 KB) - BibTeX
- EndNote
Root water uptake (RWU) is the main mechanism through which plants obtain the water they require for photosynthesis, metabolism and maintenance (McCormack et al., 2015). RWU also controls the partitioning of infiltrated soil water between groundwater recharge and local atmospheric return through evapotranspiration (Knighton et al., 2020a, b), and thus plays a key role in the global hydrological cycle. In terrestrial ecosystems, plant transpiration accounts for more than 60 % of total evapotranspiration and returns approximately 39 % of the incident precipitation to the atmosphere (Schlesinger and Jasechko, 2014; Good et al., 2015). However, although the pivotal role of RWU has long been recognized, there is limited understanding and quantification of RWU because of the opaque nature of the soil and the variability over time and space of the RWU process.
Analyses of the stable isotopes in water (δ18O and δ2H) have been extensively applied to determine the sources of water used by plants, and have provided useful insights into the RWU process (Rothfuss and Javaux, 2017; Penna et al., 2020). This application relies on the assumption that RWU is generally a nonfractionating process (Ehleringer and Dawson, 1992), so that the isotopic composition of trunk water effectively reflects that of water sources. Thus, by comparing the δ18O and δ2H values of plant trunk water to those of potential contributory water sources (e.g. water from different soil layers, groundwater and precipitation), the relative contributions of these water sources to RWU can be estimated (Rothfuss and Javaux, 2017; Wang et al., 2020; Zhao et al., 2021). However, there is a growing body of evidence that there is an isotopic offset between trunk water and potential plant water sources; that is, the isotopic composition of trunk water does not match any of the considered water sources in the dual-isotope space (Bowling et al., 2017; Vargas et al., 2017; Barbeta et al., 2019). This phenomenon has been attributed, at least in some areas, to isotopic heterogeneities among soil water pools (Oerter and Bowen, 2017; Dubbert et al., 2019). For example, isotopic data from Sprenger et al. (2019) for mobile water, bulk soil water, groundwater, stream water and derived less-mobile water over an 8-month experimental period in a Scots pine forest suggested that the mobile water and less mobile water were continuously separated. Based on a 9-month drought and rewetting experiment, Evaristo et al. (2019) found that root water uptake was mainly derived from less mobile water (89 % ± 6), not the more mobile water component in the soil matrix. The studies mentioned above rely on the assumption that there is no isotopic fractionation during RWU, but some studies indicate that such fractionation probably does contribute to the isotopic offset (Vargas et al., 2017; Barbeta et al., 2019). Lin and Sternberg (1993) and Ellsworth and Williams (2007) found evidence that hydrogen isotopic fractionation occurs during the RWU of halophytic and xerophytic plants. Poca et al. (2019) reported that arbuscular mycorrhizal fungi can enhance isotopic fractionation during RWU, resulting in up to −24.6 ‰ and −2.9 ‰ differences in δ2H and δ18O between soil and plant trunk water, respectively. In addition, the effects of extraction technology on cryogenically extracted trunk water and source water must be considered (Chen et al., 2020). Incomplete extraction of water during cryogenic distillation fractionates water stable isotopes (Gaj et al., 2017; Orlowski et al., 2018). Chen et al. (2020) found that significant isotopic deviations between cryogenically extracted trunk water and source water were common in nine woody plant species, and demonstrated that these offsets stem from methodological artefacts during cryogenic vacuum extraction. Thus, the extracted water does not properly represent the water available to plants, and may contribute to apparent trunk–soil water isotopic offsets.
It is essential to explain the isotopic offset between soil and trunk water, but it is difficult to identify the roles of specific processes due to the diversity of mechanisms that may be involved (e.g. water isotopic heterogeneities, isotopic fractionation and water extraction technology used) (Sprenger and Allen, 2020). Moreover, these mechanisms tend to have strongly interactive effects and may act on any compartment along the soil–root–trunk continuum such as the soil matrix or soil–root interface or plant woody tissues (Sprenger et al., 2019; Poca et al., 2019; Barbeta et al., 2019). Thus, it is necessary to systematically analyse the isotopic composition of each component along the pathway from soil to root to trunk. However, due to the inaccessibility of roots, much more attention has been paid to the isotopic compositions of plant trunk water and potential water sources (Chang et al., 2019; Kuhnhammer et al., 2020) than to the isotopic composition of root water (Zhao et al., 2016), leading to a lack of key information to explain observed mismatches.
Therefore, the aim of the study presented here was to analyse the hydrogen and oxygen isotopic composition of each component in the soil–root–trunk continuum. More specifically, we exploited the specific isotopic composition (δ2H and δ18O values) of mobile water, bulk soil water, groundwater and derived less mobile water to test the heterogeneity of soil water. We compared the isotopic compositions of root and soil water at the root–soil interface at 0–160 cm depths, as well as the isotopic composition of root and trunk water of Salix matsudana trees, to identify the sites and causes of the isotopic deviation more specifically. We hypothesize that soil waters with various mobilities where isotopically separated in the soil matrix, which leads to heterogeneity of the soil water, resulting in an isotopic deviation between the measured trunk water and potential water sources of S. matsudana trees during water uptake.
2.1 Site description
The study was conducted in the Liudaogou catchment (38∘47′–38∘49′ N, 110∘21′–110∘23′ E) on the Loess Plateau in China. The area and altitude of the catchment are 6.89 km2 and 1081–1274 m, respectively. The regional climate is classified as semi-arid with cool dry winters, and most of the precipitation occurs during the warm summer season. The mean annual precipitation and temperature in the catchment are 464 mm and 8.4∘, respectively. The study area received less precipitation (426 mm) than usual in the study year (2019). During this year, the seasonal distribution was uneven (70 % occurred during July to September), and the average daily temperature ranged from −13.48 ∘C in January to 26.20 ∘C in July. The Liudaogou catchment is in the water–wind erosion crisscross area of the plateau. The soil erosion modulus for this area is reportedly 15 040 t km−2 a−1 (Gong et al., 2018). Severe soil erosion has caused strongly fragmented landforms there, with gullies accounting for ca. 38 % of the total area (Zhu and Shao, 2008). Both vegetation and engineering measures (check dams) are used to mitigate soil erosion in this region. Common species used to reforest the area include Salix matsudana Koidz, S. psammophila, Caragana korshinskii and Medicago sativa L. Check dams are usually built in gullies and other channels in the area to trap runoff and sediments from steep slopes and improve agricultural yields.
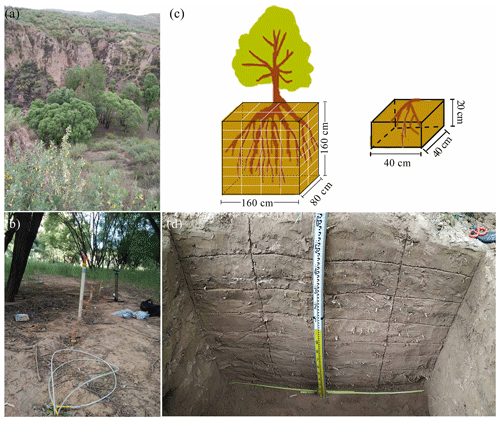
Figure 1(a) Photograph of Salix matsudana Koidz, our sampling tree. (b) Mobile water collection using suction lysimeters (white plastic tubes) (with the application of 60 kPa of tension). (c) Schematic diagram of the root excavations and measurements as described in Sect. 2.2. (d) Profile of the soil cuboid (length, width and depth: 160, 80 and 160 cm, respectively) that was dug to obtain root isotopic data. The soil cuboid was divided into 64 subcuboids, and the root isotopes in each subcuboid (length, 40 cm; width, 40 cm; height, 20 cm) were collected separately.
We selected three sampling sites in the check-dammed channel of the Liudaogou catchment. These sites were located 50, 80 and 100 m upstream of the dam and designated sites 1, 2 and 3, respectively. Salix matsudana Koidz is one of the main tree species in the check-dammed catchment, so we chose to sample S. matsudana (Fig. 1a). The average age and height of the trees were about 30 years and 12 m, respectively. The soil at the site consists of sandy loam and loam according to the USDA classification system (Table 1), with a bulk density ranging from 1.4 to 1.6 g cm−3. Water retention curves at 20, 30, 50, 100 and 150 cm soil depths at sampling site 1 are shown in Fig. S1 of the Supplement. Meteorological data on precipitation and air temperature (with a resolution of 30 min) were obtained from a weather station located about 500 m from sampling site 1. Precipitation was measured using TE525 rain gauges (Campbell Scientific Inc.), which provide ± 1 % accuracy at rates up to 25.4 mm h−1. Air temperature was measured using HMP45D probes, which have ±0.2 ∘C accuracy at 20 ∘C (Vaisala Inc.).
2.2 Measurements of roots and soil properties
We collected root samples from one S. matsudana tree and soil samples at selected soil depths (0–160 cm at 20 cm intervals) at each of the three sampling sites on 18 August 2019 to measure their isotopic composition. We excavated a soil cuboid 160 cm in depth, 80 cm in width (horizontal distance) and 160 cm in length with the main root of the selected tree at the centre (Fig. 1c–d). We then divided the cuboid into 64 subcuboids (length, 40 cm; width, 40 cm; height, 20 cm) (Fig. 1c) and dug the subcuboids one by one to minimize the risk of evaporation. Two or three coarse roots (> 2 mm in diameter) from each subcuboid were randomly selected, and roots from the top few centimetres of the topsoil were not artificially removed. To minimize the influence of the attached soil on root water, these sampled roots were rapidly peeled to remove bark before being placed in 10 mL vials sealed with caps. The caps were then secured with Parafilm. Finally, these samples were kept in a cool box until storage in the lab at 4 ∘C. To compare the isotopic compositions of root and bulk soil water at the same depths, we collected samples of soil around the sampled roots in each subcuboid. These soil samples were also rapidly placed in 10 mL vials that were sealed in the same manner as the root samples and then kept in a cool box until storage in the lab at −20 ∘C. Moreover, we collected disturbed soil samples at 10 cm intervals from 0–100 cm depths and at 20 cm intervals from 100–160 cm depths at sampling site 1 and used a soil auger to measure the soil particle size. We also collected undisturbed soil samples at 20, 30, 50, 100 and 150 cm depths using cutting rings (100 cm3 volume) to obtain water retention curves at the same sampling site. These samples were taken to the laboratory to determine their particle size using a MS 2000 laser particle size analyser (Malvern Instruments, Malvern, UK) and to obtain their water retention curves using a CR21G high-speed centrifuge (Hitachi, Japan).
2.3 Water sampling for stable isotope (δ2H and δ18O) analysis
Previously unpublished data we obtained showed that the isotopic composition of trunk water of S. matsudana trees did not match the isotopic composition of soil water in the dual-isotope space from May to September 2018 (Fig. S2). To assess the impact of soil water heterogeneity on root water uptake, we collected mobile and bulk soil water samples in 2019. Due to the effects of drought, mobile water samples could not be obtained continuously from May to July 2019 (Table S1 in the Supplement). High-frequency sampling (temporal resolution of ca. 3 d) was therefore applied to analyse the causes and locations of isotopic deviations during the period when mobile water was available (i.e. from 4 August to 15 September 2019). Soil water from 0–160 cm depths (bulk soil water, N= 247; mobile water, N= 191), groundwater (N= 22) as well as plant trunk water (N= 61) and root water (N= 156) were collected for the hydrogen and oxygen isotopic analyses. As soon as a rain event ended, precipitation samples were collected from a bottle that had a polyethylene funnel containing a plastic ball to reduce evaporation. Groundwater samples were collected at a water well located about 300 m from the soil and root sampling plot. At our study site, the mean groundwater table depth was 3.6 m, and groundwater samples were collected at ca. 30 cm depth from the surface. Soil samples were collected at 10 cm intervals from 0–100 cm depths and at 20 cm intervals from 100–160 cm depths at each of the three sampling sites (site 1, N= 68; site 2, N= 69; site 3, N= 62). These soil samples were rapidly placed in 10 mL vials and sealed with caps that were secured with Parafilm. They were then kept in a cool box until storage in the lab at −20 ∘C. The soil samples from each layer were divided into two groups: one for isotopic analysis and the other for the determination of the gravimetric soil water content (GWC, %) by the drying method (105 ∘C for 12 h). In parallel, mobile water was sampled at 20, 30, 50, 100 and 150 cm depths using suction lysimeters when water was present. Each lysimeter consisted of a porous cup with two inserted tubes that allowed the creation of a vacuum in the lysimeter and the sampling of soil water upon the injection of air into the lysimeter (Fig. 1b). A tension of 60 kPa was applied to each suction lysimeter.
Tree samples were collected simultaneously with the soil samples. These consisted of twigs collected from the south-facing side of three S. matsudana trees at a height of 250 cm on each sampling occasion. In addition, samples of trunk taken at selected tree heights (150, 250, 350, 450 cm) were collected on 18 August 2019. Bark and phloem were peeled from fully suberized branches to avoid perturbing the trunk water isotopic composition by fractionation. 30 mm long pieces of the debarked and deleaved twigs were then immediately placed in 10 mL vials, the vials were sealed with caps, and the caps were secured with Parafilm. These samples were also kept in a cool box until storage in the lab at 4 ∘C.
2.4 Stable isotope analysis
A LI-2100 automated vacuum distillation system (LICA Inc., Beijing, China) was used to extract water from the soil, trunk and root samples. This system is similar to cryogenic vacuum distillation systems that are widely used elsewhere (Gaj et al., 2017), except that it uses a compressor refrigeration unit and not liquid nitrogen. Samples were subjected to the maximum allowed vacuum pressure of 1500 Pa and a temperature differential of 225 ∘C (heating temperature, 130 ∘C; cooling trap, −95 ∘C) for 180 min during extraction to ensure that more than 99 % of the water was collected from them. The δ2H and δ18O values for all samples were determined using an Isoprime 100 stable isotope ratio mass spectrometer (Isoprime Ltd Inc., Cheadle, UK) at the Institute of Water-Saving Agriculture in Arid Areas of China, Northwest A&F University. The precision of the H and O isotopic composition analyses was 0.5 ‰ and 0.1 ‰, respectively. The isotopic composition (the 2H to 1H and 18O to 16O ratios) of the samples was normalized to the V-SMOW (Vienna Standard Mean Ocean Water) standard set by the International Atomic Energy Agency. The resulting ratios were then expressed in delta notation (δ2H and δ18O), calculated as follows:
2.5 Calculation of the lc-excess values of source water and less mobile water
We calculated the line-conditioned excess (lc-excess) values of bulk soil water, mobile water, groundwater and trunk water following Landwehr and Coplen (2006). The lc-excess values were used to identify the offset of environmental waters from precipitation. A negative lc-excess that exceeds the standard deviation of the local meteoric water line (LMWL) indicates that the water has undergone evaporative isotopic enrichment (Evaristo et al., 2016). The lc-excess values of the samples were calculated as follows:
where the subscript s refers to the sample and a and b are the slope and intercept of the LMWL, respectively. The LMWL shows the relationship between δ2H and δ18O in precipitation; according to an analysis of the precipitation (N= 89) from 2016 to 2019 at our study site, this relationship was δ2H = 7.67 δ18O + 5.91.
In addition, following Sprenger et al. (2019), we determined the maximum value of less mobile water (here defined as water that could not be accessed by a suction lysimeter) at selected depths (20, 30, 50, 100 and 150 cm); that is, the GWC determined by the application of 60 kPa of suction (the tension applied to obtain mobile water). The mobile fraction of soil water was calculated as the difference between the measured bulk soil water and the less mobile water. Based on an isotope mass balance approach, the isotopic composition of less mobile water was calculated as follows:
Here, the δ and θ parameters represent the isotopic compositions and GWCs of the samples, respectively, while the subscripts LMW, BW and MW represent the less mobile water, bulk soil water and mobile water, respectively.
2.6 Statistical analysis
All statistical analyses were performed using SPSS 20.0 (SPSS Inc., Chicago, USA). Shapiro–Wilk and Levene's tests were respectively used to check that the data met the normality of distribution and homogeneity of variance requirements for the planned analyses. One-way ANOVA followed by Tukey's test was used to detect significant differences in variation in depth between the less mobile water, root water, mobile water, and bulk soil water isotopic compositions. Presented diagrams were generated using SigmaPlot 12.5.
3.1 Dual-isotope plots
The stable isotopic compositions (δ2H and δ18O) of all the water samples are shown in Fig. 2a and Table 2. The slope and intercept of the local meteoric water line (LMWL, δ2H = 7.67 δ18O + 5.91, R2= 0.96) were lower than those of the global meteoric water line (GMWL, δ2H = 8 δ18O + 10) (Craig, 1961). The data points for mobile water at all depths (i.e. 20, 30, 50, 100 and 150 cm) were typically situated on the LMWL, and groundwater was isotopically similar to mobile water at 150 cm depth (Fig. 2b). Bulk soil water partly overlapped isotopically with mobile water, but it was generally plotted below mobile water (Fig. 2a and c). Less mobile water deviated from the LMWL and overlapped with root water and trunk water (Fig. 2a and d). Trunk water was isotopically similar to root water at 100–160 cm depths (Fig. 2a and e–f).
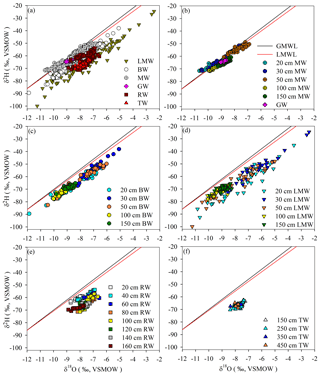
Figure 2(a) δ18O and δ2H isotopic compositions collected from 4 August to 15 September 2019. Plotted values include bulk soil water (BW), mobile water (MW), root water (RW), trunk water (TW), less mobile water (LMW) and groundwater (GW). The other plots show the δ18O and δ2H isotopic compositions of (b) GW and MW collected from different depths, (c) BW collected from different depths, (d) LMW collected from different depths, (e) RW collected from different depths, and (f) TW collected at different tree heights. The red line represents the 2016–2019 local meteoric water line (LMWL, δ2H = 5.91 + 7.67 δ18O, R2= 0.96). The black line represents the global meteoric water line (GMWL, δ2H = 10 + 8 δ18O).
Table 2Stable isotopes in water (see Fig. 2) and lc-excess values (Fig. 3) for all water samples. Range values show minimum, maximum (mean).
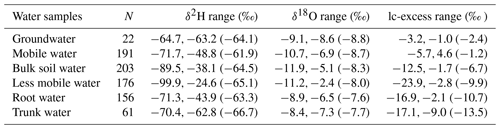
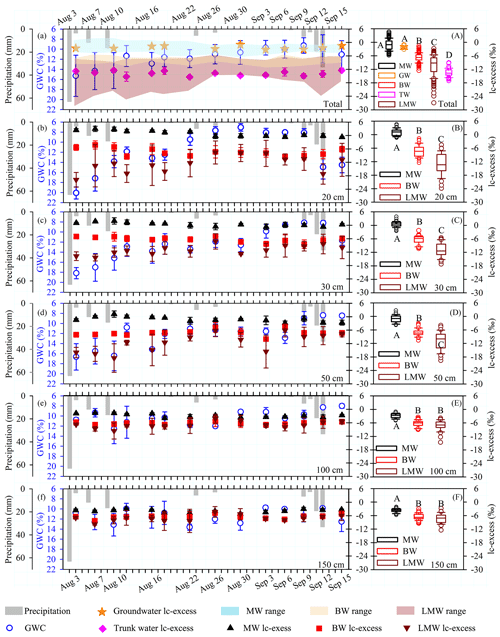
Figure 3(a–f) Temporal dynamics of hydrological conditions (precipitation and gravimetric water content, GWC) and lc-excess values (these values are means and standard deviations based on three sites) of groundwater (GW), trunk water (TW), mobile water (MW), bulk soil water (BW) and less mobile water (LMW) at the indicated depths (20, 30, 50, 100 and 150 cm) during the period 3 August to 15 September 2019. (A) Boxplots of total MW (N= 191), GW (N= 22), BW (N= 204), TW (N= 61) and LMW (N= 176) lc-excess values. (B–F) Boxplots of MW and BW at depths of 20 cm (MW, N= 40; BW, N= 42; LMW, N= 39), 30 cm (MW, N= 40; BW, N= 40; LMW, N= 34), 50 cm (MW, N= 38; BW, N= 40; LMW, N= 33), 100 cm (MW, N= 36; BW, N= 40; LMW, N= 34) and 150 cm (MW, N= 37; BW, N= 42; LMW, N= 36). The top and bottom of each box are the 25th and 75th percentiles of the samples, respectively. The black line in each box is the sample median. Trunk water and potential water sources that do not share a letter are significantly different (p < 0.05, Tukey–Kramer HSD).
3.2 The lc-excess values of mobile water, bulk soil water and less mobile water
As shown in Fig. 3a and Table 2, the mean lc-excess values of groundwater and mobile water did not significantly differ (p > 0.05), and they were significantly higher than those of bulk soil water, less mobile water and trunk water (Tukey–Kramer HSD, p < 0.05) during the sampling period (4 August to 15 September 2019). The lc-excess of trunk water was generally lower than that of bulk soil water but within the range of lc-excess values for less mobile water. A heavy rain event occurred the day before the sampling (3 August), with 63 mm of precipitation. The shallow GWC (at 20–30 cm) was sensitive to this rain event and decreased gradually from 4 August to 9 September (Fig. 3b–c). Although GWC varied greatly, mobile water and bulk soil water at 20–30 cm depths remained relatively stable during this period, with average lc-excess values of 0.9±1.1 ‰ and −6.8 ± 1.6 ‰, respectively. The lc-excess values of less mobile water at the same depths gradually increased and stabilized, ranging from −23.9 ‰ to −4.6 ‰. The GWC at deep layers (i.e. 100 and 150 cm) was less affected by precipitation, ranging from 8.0 % to 13.6 %. Similarly, the mean lc-excess values of mobile water, bulk soil water and less mobile water in the 100–150 cm layer fluctuated slightly from 4 August to 9 September, with average values of −3.3 ± 1.1 ‰, −6.5 ± 1.4 ‰ and −7.4 ± 2.1 ‰, respectively (Fig. 3e–f).
At every sampling depth, the mean lc-excess of mobile water was always higher than that of bulk soil water and less mobile water (Tukey–Kramer HSD, p < 0.05) during the whole sampling period (Fig. 3b–f). In particular, the most significant difference between mobile water, bulk soil water and less mobile water appeared in the 20 cm soil layer, with average lc-excess values of 1.1 ± 1.5 ‰, −7.3 ± 2.5 ‰ and −12.8 ± 4.3 ‰, respectively. No correlation between Δ lc-excess (the lc-excess difference between the measured mobile water and bulk soil water) and GWC was detected at 20–150 cm depths, but a strong positive correlation between lc-excess value and GWC was observed at 20, 30 and 50 cm depths for mobile water (20 cm, y= 0.19x−1.27, R2= 0.27, N= 40, p= 0.001; 30 cm, y= 0.17x−1.61, R2= 0.22, N= 40, p= 0.002; 50 cm, y= 0.20x−3.59, R2= 0.16, N= 38, p= 0.013) and at 20–30 cm depths for bulk soil water (20 cm, y= 0.34x−11.39, R2= 0.30, N= 42, p < 0.001; 30 cm, y= 0.23x−9.21, R2= 0.20, N= 40, p= 0.003) (Fig. 4a–c). No correlation between these variables was detected (Fig. 4d–e) at 100 and 150 cm depths for mobile water and bulk soil water.
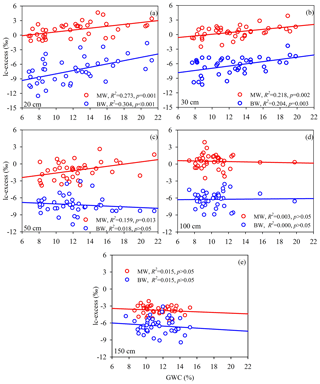
Figure 4Relationships between gravimetric water content (GWC) and (a) lc-excess values at 20 cm depth, (b) lc-excess values at 30 cm depth, (c) lc-excess values at 50 cm depth, (d) lc-excess values at 100 cm depth and (e) lc-excess values at 150 cm depth. Values of lc-excess for mobile water (MW) and bulk soil water (BW) are shown as red and blue circles, respectively. The insets summarize the goodness of fit of the linear regressions.
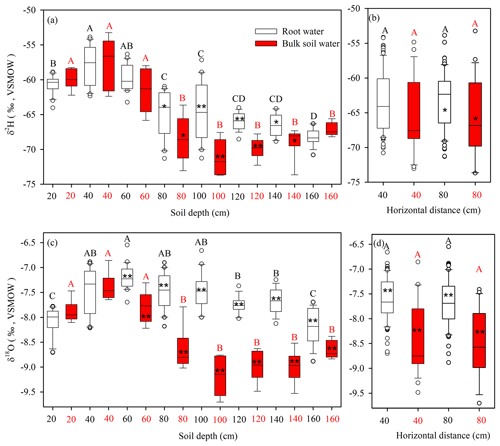
Figure 5Boxplots of stable isotope compositions of root water and bulk soil water (δ2H and δ18O) at indicated depths (a, c), and horizontal distances from the tap root of the focal root system (b, d). The top and bottom of each box are the 25th and 75th percentiles of the samples, respectively. The black line in each box is the sample median. Asterisks indicate significantly different isotopic compositions of soil water and root water (* and p < 0.05 and p < 0.01, respectively, according to two-tailed tests). Stable isotope compositions of plant root water or bulk soil water at different depths that do not share a letter are significantly different (p < 0.05, Tukey–Kramer HSD).
3.3 Comparison between root water and bulk soil water isotopic composition at different depths
As shown in Fig. 5b and d, there were no significant differences (p > 0.05) between the isotopic compositions (δ2H and δ18O) of root water or bulk soil water at horizontal distances of 40 and 80 cm from selected tree trunks, suggesting that the isotopic composition of the bulk soil water presented little horizontal variation within 80 cm from the taproots. However, isotopic variations with depth were detected for both root water and bulk soil water. Generally higher δ2H and δ18O values were observed for root water (mean values and standard deviations for three soil profiles: −65.90 ± 2.92 ‰ and −7.66 ± 0.40 ‰, respectively) than for bulk soil water (mean values and standard deviations for three soil profiles: −69.09 ± 2.50 ‰ and −8.89 ± 0.38 ‰, respectively) at 80–160 cm depths. Root water and bulk soil water significantly differed in δ2H at 80–140 cm depths (p < 0.05) and in δ18O at 60–160 cm depths (p < 0.01) (Fig. 5a and c). The maximum differences in Δ2H and Δ18O between bulk soil water and root water were −8.6 ‰ and −1.8 ‰, respectively. Although the δ2H and δ18O values of root water and bulk soil water behaved differently, a strong correlation was observed between Δ18O (Δ18O =δ18Osoil−δ18Oroot) and Δ2H (Δ2H =δ2Hsoil−δ2Hroot) for the soil–root offset (Fig. 6a) at 0–160 cm depths (bulk soil water–root water: , R2= 0.69, N= 24, p < 0.001). Similarly, a strong correlation was observed between the Δ18O (Δ18O =δ18Osoil−δ18Otrunk) and Δ2H (Δ2H = δ2Hsoil−δ2Htrunk) soil–trunk offsets during 4 August to 15 September (bulk soil water–trunk water: , R2= 0.83, N= 42, p < 0.001; mobile water–trunk water: , R2= 0.81, N= 42, p < 0.001) (Fig. 6b).
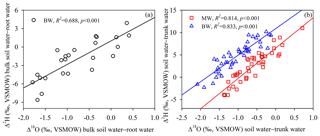
Figure 6(a) Relationship of the hydrogen isotope offset (Δ2H, Δ2H =δ2Hsoil−δ2Hroot) to the oxygen isotope offset (Δ18O, Δ18O =δ18Osoil−δ18Oroot) for comparisons between bulk soil water and root water, according to analyses of samples of bulk soil water (BW) and root water collected from 0–160 cm depths on 18 August 2019. (b) Relationship of the hydrogen isotope offset (Δ2H, Δ2H =δ2Hsoil−δ2Htrunk) to the oxygen isotope offset (Δ18O, Δ18O =δ18Osoil−δ18Otrunk) for comparisons between soil water and trunk water, according to analyses of samples of bulk soil water, mobile water (MW) and trunk water collected from 4 August to 15 September 2019. The insets summarize the goodness of fit of the linear regressions (a–b).
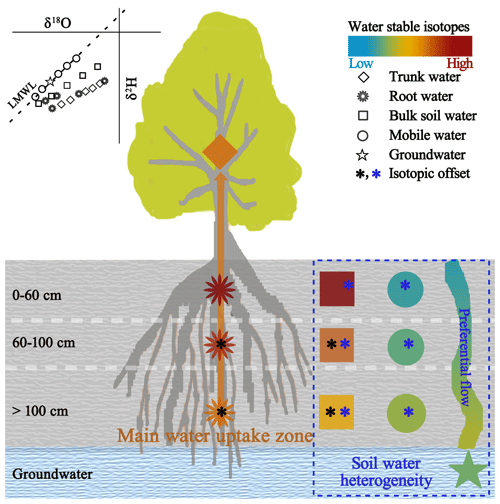
Figure 7Schematic diagram of isotopic dynamics along the soil–root–trunk continuum. Colour codes indicate the isotopic compositions of mobile water, bulk soil water and root water at the indicated depths, as well as those of groundwater and trunk water (colours range from from blue to brown, representing low to high values). The upper left inset is a conceptual dual-isotope plot. A black asterisk indicates a significant difference in isotopic offset between root water and bulk soil water at the same depth (p < 0.05). A blue asterisk indicates a significant difference in isotopic offset between mobile water and bulk soil water at the same depth (p < 0.05).
4.1 Isotopic dynamics at the root–soil interface
4.1.1 Separation of mobile water and bulk soil water in the soil matrix
At our study site during the experimental period (4 August to 15 September 2019), a clear isotopic separation between mobile and bulk soil water was observed (Figs. 3 and 7). A key question is: why does mobile water separate from bulk soil water isotopically? Gierke et al. (2016) examined the stable isotopic composition of precipitation, bulk soil water and trunk water in a high-elevation watershed, and their results suggested that mobile water was primarily associated with summer thunderstorms and thus subject to minimal evaporative loss. In contrast, less mobile water was derived from snowmelt that filled small pores in the shallow soils. Allen et al. (2019) characterized the occurrence of winter and summer precipitation in plant trunk samples using a seasonal origin index and found that winter precipitation was the predominant water source for midsummer transpiration in sampled beech and oak trees. Due to seasonal isotopic cycles in precipitation, the difference in isotopic composition between mobile water and less mobile water derived from precipitation may vary significantly over time (Bowen et al., 2019). At our study site, precipitation in winter (December–February) and summer (June–September) accounted for 2 % and 77 % of the total average annual precipitation (464 mm) from 2003 to 2019, respectively. Such small amounts of winter precipitation might not be able to fill the small pores. Notably, there was a major rainstorm the day before the sampling (3 August), with 63 mm of precipitation. The mean GWC in the 0–50 and 100–150 cm layers reached 17.4 ± 2.7 % and 10.8 ± 1.5 % between 4 and 7 August, respectively. These results imply that precipitation greatly supplemented the water in the upper soil layer. Therefore, mobile water collected by suction lysimeters during this period contained a considerable proportion of water from the rain event on 3 August. In contrast, bulk soil water contained not only mobile water from this rain event but also antecedent less-mobile water that could not be extracted by a suction lysimeter, resulting in the isotopic separation between mobile water and bulk soil water. Furthermore, the lc-excess values of both mobile and bulk soil water were positively correlated with the GWC at 20 and 30 cm depths. When GWC increased due to precipitation, the lc-excess values of mobile and bulk soil water increased. Similarly, when the GWC decreased due to evaporation, those lc-excess values also decreased. The lc-excess values of mobile and bulk soil water consistently differed significantly, although the GWC varied greatly, suggesting a clear isotopic separation between mobile and bulk soil water that is not affected by the GWC. This result is consistent with the finding by Evaristo et al. (2016) that ecohydrological separation was consistently present in two tropical catchments with contrasting moisture conditions (the Luquillo and Susua catchments in Puerto Rico, with mean annual precipitations of 3700 and 1200 mm, respectively).
We also found that the degree of separation between the lc-excess values of mobile and bulk soil water gradually decreased as the soil depth increased (e.g. to 100 and 150 cm). On the one hand, the effect of soil evaporation on bulk soil water gradually weakens with increasing soil depth. Thus, the isotopic composition enrichment caused by the evaporation of bulk soil water gradually declines or even disappears. On the other hand, mobile water in deep layers is more likely to be recharged by both preferential and matrix flows than by preferential flow alone (Xiang et al., 2019). Under matrix flow conditions, newly infiltrated water displaces existing `old' water, pushing it deeper into the soil profile and eventually into groundwater (Zheng et al., 2019), so both the mobile water and the less mobile water in deep layers are more fully mixed than they are in shallow layers (Sprenger et al., 2016; Kubert et al., 2020). Evidence of this mixing has been provided by Vargas et al. (2017), who found that 75–95 % of the less mobile water isotopically exchanged with mobile water in a glasshouse experiment with Persea americana potted in two contrasting soil types. In addition, Adams et al. (2020) found that the isotopic compositions of mobile and less mobile water are affected by the soil texture and mineralogy (e.g. the smectite and clay contents). The extent to which less mobile water mixes with mobile water at our study site is unclear, but such exchange might be one of the reasons for the weakening of the separation between mobile and bulk soil water in deep layers.
4.1.2 Isotopic offset between bulk soil water and root water
We compared the isotopic compositions of root water and bulk soil water at the same depth (Fig. 5). Contrary to expectations, the root water and bulk soil water at 0–60 cm depths showed consistent δ2H and δ18O values. However, at 80–160 cm depths, the δ2H and δ18O values of root water deviated significantly from those of bulk soil water. An alternative explanation for the isotopic mismatch at these depths is that it is due to the complexity of root systems and difficulties in unambiguously determining root traits and functions at specific depths because of the opaque nature of the soil. For example, if collected roots are close to absorptive roots, such as fine roots (< 2 mm in diameter), they may have a similar isotopic composition to bulk soil water at the same depth. In contrast, if they are closer to transport roots such as taproots, much of their water content may be from different positions, thereby resulting in inconsistent isotopic compositions of the root water and surrounding bulk soil water. Nevertheless, although it is difficult to assess the similarity of sampled root water to the whole root system's water uptake, root water may reflect the water sources of trees better than bulk soil water (which has been used more extensively) for two reasons. First, bulk soil water is commonly collected in cores of 50 cm3 or more (Sprenger et al., 2015; Penna et al., 2018). It is possible to determine the fractions and isotopic composition of bulk soil water held under specific tension ranges, but information on the spatiotemporal heterogeneity of pore sizes within the cores and associated effects on uptake patterns is lost (McCutcheon et al., 2016). Root water is not subject to this deficiency as it consists of water absorbed by fine roots distributed in pores of various sizes. In addition, we systematically collected coarse roots (> 2 mm in diameter) within 80 cm of the main trunk at 20 cm intervals in the 0–160 cm deep soil layer to reduce the potential errors caused by the lack of representativeness of some root water. Our results suggest that trunk water was isotopically closer to root water than to bulk soil water. Similarly, measurements by Barbeta et al. (2020) of the δ2H and δ18O for soil, trunk and root water from potted Fagus sylvatica saplings under control and drought treatments showed that the δ2H of trunk water consistently matched the δ2H of root water and deviated significantly from the δ2H of soil water under both treatments.
Overall, the most plausible explanation for the isotopic mismatch between root water and bulk soil water in dual-isotope plots is that bulk soil water is not representative of the available plant water sources because of the heterogeneity of bulk soil water. As shown in Fig. 2a, less mobile water overlapped isotopically with root water after removing the influence of mobile water. The speed at which mobile water passes through soil reduces its contact with mineral surfaces and hence its nutrient concentrations (McDonnell, 2017; Sprenger et al., 2019). Thus, in the present study, plants may have used large amounts of less mobile water that was strongly affected by evaporative effects. This less mobile water is isotopically distinct from mobile water and groundwater and has a similar isotopic composition to trunk water. In addition, isotopic offsets between bulk soil water and root/trunk water caused by isotopic fractionation have been reported (Lin and Sternberg, 1993; Vargas et al., 2017; Barbeta et al., 2019). Vargas et al. (2017) found that isotopic fractionation caused more 2H depletion in trunk water than in bulk soil water. Similarly, Poca et al. (2019) found that trunk water was significantly more depleted in 2H than bulk soil water (by up to −15.6 ‰), and that this isotopic fractionation occurred during transmembrane water transport by aquaporins. However, these findings are not consistent with the greater 2H enrichment in root water than in bulk soil water (differences of up to 8.6 ‰) we detected, suggesting that soil–root isotopic offsets are more likely to be caused by the complexity of root systems and heterogeneity of bulk soil water than isotopic fractionation during root water uptake.
4.2 Root water and trunk water isotopic compositions
We found that trunk water mainly overlapped isotopically with root water at 100–160 cm depths (Fig. 2a and e–f), while the isotopically enriched root water at 0-80 cm depths was not reflected in the trunk water isotopic composition. As the time required for the isotopic tracer (D2O) to move from the base of a trunk to the upper crown of a tree reportedly ranges from 2.5 to 21 d (Meinzer et al., 2016), the isotopic composition of trunk water may differ from that root water collected on the same day (18 August). We thus measured δ2H and δ18O values of trunk water during our high-frequency (ca. 3 d) sampling period from 4 August to 15 September 2019 (Fig. 3a) and found that the δ2H and δ18O values of trunk water remained stable (mean values: −66.68 ± 1.61 and −7.71 ± 0.24 ‰, respectively) during this period. Moreover, to test the possibility that the isotopic composition of trunk water may be heterogeneous at different tree heights, we collected trunk water at 150–450 cm tree heights on 18 August 2019, and found no significant differences in isotopic composition (p > 0.05) (Fig. S3). These results indicated that the trees always used a stable water source during the study period. One possibility is that trees preferentially use much deeper soil water and groundwater than fluctuating shallow soil water, which is a less stable and reliable water source because it is subject to rapid evaporation and seasonal precipitation (Zhao and Wang, 2018). Deep soil water can make a significant contribution to drought avoidance during dry periods (Yang et al., 2017), and an increasing capacity for deep soil water utilization was positively correlated with intrinsic water use efficiency (Jiang et al., 2020). Moreover, the deep water use strategy of S. matsudana may provide favourable water conditions for shallow-rooted herbaceous species, facilitating stable coexistence. Roots at 0–80 cm depths absorb less water with an enriched isotopic composition than deep roots. A small proportion of the isotopically enriched root water fully mixes with isotopically depleted root water in deep layers, resulting in the disappearance of isotopically enriched signals from the trunk water. Furthermore, previous studies have provided indications that trunk water becomes more enriched in 18O due to declines in sap flow rates over time (Martin-Gomez et al., 2017) and the mixing of trunk water with leaf water (Brandes et al., 2007). However, we did not find that the trunk water of the trees we sampled had higher 18O values than root water (Fig. 2a and e–f). Therefore, we believe that the partial overlap in isotopic composition between root water and trunk water seen in this study reflects the selective utilization of water sources rather than isotopic fractionation within woody tissues.
At our study site during the experimental period, there was an isotopic offset between trunk water of S. matsudana trees and bulk soil water. We explored the causes of this mismatch and the sources of water taken up by the trees by analysing the stable isotope compositions of soil waters with various mobilities, root water and trunk water. In the soil matrix, bulk soil water generally had lower lc-excess values than mobile water due to the effects of soil evaporation and the mixing of newly infiltrated mobile and less mobile water with increasing depth. The isotopic composition of root water did not fully match that of bulk soil water at the same depth due to the complexity of root systems and soil water heterogeneity. The maximum differences in δ2H and δ18O between bulk soil water and root water were −8.6 ‰ and −1.8 ‰, respectively. Overall, the δ2H and δ18O values derived for less mobile water overlapped with those of root water and trunk water, and the trunk water values mainly overlapped with those of root water at 100–160 cm depths. These findings suggest that the isotopic offset between bulk soil water and trunk water was due to an isotopic mismatch between root water and bulk soil water associated with the heterogeneity of the soil water. The presented stable isotope data for bulk soil water, mobile water, less mobile water, root water and trunk water were highly valuable for analysing the spatial heterogeneity of water fluxes in the root zone and elucidating the water sources used by the plants.
The data that support the findings of this study are available from the corresponding author upon request.
The supplement related to this article is available online at: https://doi.org/10.5194/hess-25-3975-2021-supplement.
LW conceptualized this research. YZ collected the data. Both authors contributed to the writing of the manuscript.
The authors declare that they have no conflict of interest.
Publisher’s note: Copernicus Publications remains neutral with regard to jurisdictional claims in published maps and institutional affiliations.
We thank Ziqi Yang, Chaohui Wei, Jinxia An and Zhoufeng Li for their support during field work. We also wish to thank the anonymous reviewers and the handling editor, Matthias Sprenger, for their positive feedback and helpful suggestions, which improved the manuscript.
This research has been supported by the National Natural Science Foundation of China (grant nos. 41771545 and 41977012), the Strategic Priority Research Program of the Chinese Academy of Sciences (grant no. XDB40000000), and the State Key Laboratory of Loess and Quaternary Geology, Institute of Earth Environment, CAS (ref. no. SKLLQG1718).
This paper was edited by Matthias Sprenger and reviewed by three anonymous referees.
Adams, R. E., Hyodo, A., SantaMaria, T., Wright, C. L., Boutton, T. W., and West, J. B.: Bound and mobile soil water isotope ratios are affected by soil texture and mineralogy, whereas extraction method influences their measurement, Hydrol. Process., 34, 991–1003, 2020.
Allen, S. T., Kirchner, J. W., Braun, S., Siegwolf, R. T. W., and Goldsmith, G. R.: Seasonal origins of soil water used by trees, Hydrol. Earth Syst. Sci., 23, 1199–1210, https://doi.org/10.5194/hess-23-1199-2019, 2019.
Barbeta, A., Jones, S. P., Clavé, L., Wingate, L., Gimeno, T. E., Fréjaville, B., Wohl, S., and Ogée, J.: Unexplained hydrogen isotope offsets complicate the identification and quantification of tree water sources in a riparian forest, Hydrol. Earth Syst. Sci., 23, 2129–2146, https://doi.org/10.5194/hess-23-2129-2019, 2019.
Barbeta, A., Gimeno, T. E., Clave, L., Frejaville, B., Jones, S. P., Delvigne, C., Wingate, L., and Ogee, J. M.: An explanation for the isotopic offset between soil and stem water in a temperate tree species, New Phytol., 227, 766–779, 2020.
Bowen, G. J., Cai, Z. Y., Fiorella, R. P., and Putman, A. L.: Isotopes in the water cycle: regional- to global-scale patterns and applications, Annu. Rev. Earth Pl. Sci., 47, 453–479, 2019.
Bowling, D. R., Schulze, E. S., and Hall, S. J.: Revisiting streamside trees that do not use stream water: can the two water worlds hypothesis and snowpack isotopic effects explain a missing water source?, Ecohydrology, 10, e1771, https://doi.org/10.1002/eco.1771, 2017.
Brandes, E., Wenninger, J., Koeniger, P., Schindler, D., Rennenberg, H., Leibundgut, C., Mayer, H., and Gessler, A.: Assessing environmental and physiological controls over water relations in a Scots pine (Pinus sylvestris L.) stand through analyses of stable isotope composition of water and organic matter, Plant Cell Environ., 30, 113–127, 2007.
Chang, E. H., Li, P., Li, Z. B., Xiao, L., Zhao, B. H., Su, Y. Y., and Feng, Z. H.: Using water isotopes to analyze water uptake during vegetation succession on abandoned cropland on the Loess Plateau, China, Catena, 181, 104095, https://doi.org/10.1016/j.catena.2019.104095, 2019.
Chen, Y. L., Helliker, B. R., Tang, X. H., Li, F., Zhou, Y. P., and Song, X.: Stem water cryogenic extraction biases estimation in deuterium isotope composition of plant source water, P. Natl. Acad. Sci. USA, 117, 33345–33350, https://doi.org/10.1073/pnas.2014422117, 2020.
Craig, H.: Isotopic variations in meteoric waters, Science, 133, 1702–1703, 1961.
Dubbert, M., Caldeira, M. C., Dubbert, D., and Werner, C.: A pool-weighted perspective on the two-water-worlds hypothesis, New Phytol., 222, 1271–1283, 2019.
Ehleringer, J. R. and Dawson, T. E.: Water uptake by plants: perspectives from stable isotope composition, Plant Cell Environ., 15, 1073–1082, 1992.
Ellsworth, P. Z. and Williams, D. G.: Hydrogen isotope fractionation during water uptake by woody xerophytes, Plant Soil, 291, 93–107, 2007.
Evaristo, J., McDonnell, J. J., Scholl, M. A., Bruijnzeel, L. A., and Chun, K. P.: Insights into plant water uptake from xylem-water isotope measurements in two tropical catchments with contrasting moisture conditions, Hydrol. Process., 30, 3210–3227, 2016.
Evaristo, J., Kim, M., van Haren, J., Pangle, L. A., Harman, C. J., Troch, P. A., and McDonnell, J. J.: Characterizing the fluxes and age distribution of soil water, plant water and deep percolation in a model tropical ecosystem, Water Resour. Res., 55, 3307–3327, 2019.
Gaj, M., Kaufhold, S., Koeniger, P., Beyer, M., Weiler, M., and Himmelsbach, T.: Mineral mediated isotope fractionation of soil water, Rapid Commun. Mass Sp., 31, 269–280, 2017.
Gierke, C., Newton, B. T., and Phillips, F. M.: Soil-water dynamics and tree water uptake in the Sacramento Mountains of New Mexico (USA): a stable isotope study, Hydrogeol. J., 24, 805–818, 2016.
Gong, T. X., Zhu, Y. J., and Shao, M. A.: Spatial distribution of caliche nodules in surface soil and their influencing factors in the Liudaogou catchment of the northern Loess Plateau, China, Geoderma, 329, 11–19, 2018.
Good, S. P., Noone, D., and Bowen, G.: Hydrologic connectivity constrains partitioning of global terrestrial water fluxes, Science, 349, 175–177, 2015.
Jiang, P. P., Wang, H. M., Meinzer, F. C., Kou, L., Dai, X. Q., and Fu, X. L.: Linking reliance on deep soil water to resource economy strategies and abundance among coexisting understory shrub species in subtropical pine plantations, New Phytol., 225, 222–233, 2020.
Knighton, J., Kuppel, S., Smith, A., Soulsby, C., Sprenger, M., and Tetzlaff, D.: Using isotopes to incorporate tree water storage and mixing dynamics into a distributed ecohydrologic modelling framework, Ecohydrology, 13, e2201, https://doi.org/10.1002/eco.2201, 2020a.
Knighton, J., Singh, K., and Evaristo, J.: Understanding catchment-ccale forest root water uptake strategies across the continental United States through inverse ecohydrological modeling, Geophys. Res. Lett., 47, e2019GL085937, https://doi.org/10.1029/2019GL085937, 2020b.
Kubert, A., Paulus, S., Dahlmann, A., Werner, C., Rothfuss, Y., Orlowski, N., and Dubbert, M.: Water stable isotopes in ecohydrological field research: comparison between in situ and destructive monitoring methods to determine soil water isotopic signatures, Front. Plant Sci., 11, 387, https://doi.org/10.3389/fpls.2020.00387, 2020.
Kuhnhammer, K., Kubert, A., Bruggemann, N., Diaz, P. D., van Dusschoten, D., Javaux, M., Merz, S., Vereecken, H., Dubbert, M., and Rothfuss, Y.: Investigating the root plasticity response of Centaurea jacea to soil water availability changes from isotopic analysis, New Phytol., 226, 98–110, 2020.
Landwehr, J. M. and Coplen, T. B.: Line-conditioned excess: a new method for characterizing stable hydrogen and oxygen isotope ratios in hydrologic systems, in: International Conference on Isotopes in Environmental Studies, Aquatic Forum, Monte-Carlo, Monaco, 25–29 October 2004, IAEA, Vienna, 132–135, 2006.
Lin, G. H. and Sternberg, L. da S. L.: Hydrogen isotopic fractionation by plant roots during water uptake in coastal wetland plants, in: Stable isotopes and plant carbon-water relations, edited by: Ehleringer, J., Hall, A., and Farquhar, G., Academic Press Inc., New York, 497–510, 1993.
Martin-Gomez, P., Serrano, L., and Ferrio, J. P.: Short-term dynamics of evaporative enrichment of xylem water in woody stems: implications for ecohydrology, Tree Physiol., 37, 511–522, 2017.
McCormack, M. L., Dickie, I. A., Eissenstat, D. M., Fahey, T. J., Fernandez, C. W., Guo, D. L., Helmisaari, H. S., Hobbie, E. A., Iversen, C. M., Jackson, R. B., Leppalammi-Kujansuu, J., Norby, R. J., Phillips, R. P., Pregitzer, K. S., Pritchard, S. G., Rewald, B., and Zadworny, M.: Redefining fine roots improves understanding of below-ground contributions to terrestrial biosphere processes, New Phytol., 207, 505–518, 2015.
McCutcheon, R. J., McNamara, J. P., Kohn, M. J., and Evans, S. L.: An evaluation of the ecohydrological separation hypothesis in a semiarid catchment, Hydrol. Process. 31, 1–17, 2016.
McDonnell, J. J.: Beyond the water balance, Nat. Geosci., 10, 396–396, 2017.
Meinzer, F. C., Woodruff, D. R., Marias, D. E., Smith, D. D., Mcculloh, K. A., Howard, A. R., and Magedman, A. L.: Mapping “hydroscapes” along the iso- to anisohydric continuum of stomatal regulation of plant water status, Ecol. Lett., 19, 1343–1352, 2016.
Oerter, E. J. and Bowen, G.: In situ monitoring of H and O stable isotopes in soil water reveals ecohydrologic dynamics in managed soil systems, Ecohydrology, 10, e1841, https://doi.org/10.1002/eco.1841, 2017.
Orlowski, N., Breuer, L., Angeli, N., Boeckx, P., Brumbt, C., Cook, C. S., Dubbert, M., Dyckmans, J., Gallagher, B., Gralher, B., Herbstritt, B., Hervé-Fernández, P., Hissler, C., Koeniger, P., Legout, A., Macdonald, C. J., Oyarzún, C., Redelstein, R., Seidler, C., Siegwolf, R., Stumpp, C., Thomsen, S., Weiler, M., Werner, C., and McDonnell, J. J.: Inter-laboratory comparison of cryogenic water extraction systems for stable isotope analysis of soil water, Hydrol. Earth Syst. Sci., 22, 3619–3637, https://doi.org/10.5194/hess-22-3619-2018, 2018.
Penna, D., Hopp, L., Scandellari, F., Allen, S. T., Benettin, P., Beyer, M., Geris, J., Klaus, J., Marshall, J. D., Schwendenmann, L., Volkmann, T. H. M., von Freyberg, J., Amin, A., Ceperley, N., Engel, M., Frentress, J., Giambastiani, Y., McDonnell, J. J., Zuecco, G., Llorens, P., Siegwolf, R. T. W., Dawson, T. E., and Kirchner, J. W.: Ideas and perspectives: Tracing terrestrial ecosystem water fluxes using hydrogen and oxygen stable isotopes – challenges and opportunities from an interdisciplinary perspective, Biogeosciences, 15, 6399–6415, https://doi.org/10.5194/bg-15-6399-2018, 2018.
Penna, D., Geris, J., Hopp, L., and Scandellari, F.: Water sources for root water uptake: Using stable isotopes of hydrogen and oxygen as a research tool in agricultural and agroforestry systems, Agr. Ecosyst. Environ., 291, 106790, https://doi.org/10.1016/j.agee.2019.106790, 2020.
Poca, M., Coomans, O., Urcelay, C., Zeballos, S. R., Bode, S., and Boeckx, P.: Isotope fractionation during root water uptake by Acacia caven is enhanced by arbuscular mycorrhizas, Plant Soil, 441, 485–497, 2019.
Rothfuss, Y. and Javaux, M.: Reviews and syntheses: Isotopic approaches to quantify root water uptake: a review and comparison of methods, Biogeosciences, 14, 2199–2224, https://doi.org/10.5194/bg-14-2199-2017, 2017.
Schlesinger, W. H. and Jasechko, S.: Transpiration in the global water cycle, Agr. Forest Meteorol., 189–190, 115–117, 2014.
Sprenger, M. and Allen, S. T.: What ecohydrologic separation is and where we can go with it, Water Resour. Res., 56, e2020WR027238, https://doi.org/10.1029/2020WR027238, 2020.
Sprenger, M., Herbstritt, B., and Weiler, M.: Established methods and new opportunities for pore water stable isotope analysis, Hydrol. Process., 29, 5174–5192, 2015.
Sprenger, M., Leistert, H., Gimbel, K., and Weiler, M.: Illuminating hydrological processes at the soil-vegetation-atmosphere interface with water stable isotopes, Rev. Geophs., 54, 674–704, 2016.
Sprenger, M., Llorens, P., Cayuela, C., Gallart, F., and Latron, J.: Mechanisms of consistently disjunct soil water pools over (pore) space and time, Hydrol. Earth Syst. Sci., 23, 2751–2762, https://doi.org/10.5194/hess-23-2751-2019, 2019.
Vargas, A. I., Schaffer, B., Yuhong, L., and Sternberg, L. S. L.: Testing plant use of mobile vs immobile soil water sources using stable isotope experiments, New Phytol., 215, 582–594, 2017.
Wang, J., Fu, B. J., Wang, L. X., Lu, N., and Li, J. Y.: Water use characteristics of the common tree species in different plantation types in the Loess Plateau of China, Agr. Forest Meteorol., 288–289, 108020, https://doi.org/10.1016/j.agrformet.2020.108020, 2020.
Xiang, W., Si, B. C., Biswas, A., and Li, Z.: Quantifying dual recharge mechanisms in deep unsaturated zone of Chinese Loess Plateau using stable isotopes, Geoderma, 337, 773–781, 2019.
Yang, F. T., Feng, Z. M., Wang, H. M., Dai, X. Q., and Fu, X. L.: Deep soil water extraction helps to drought avoidance but shallow soil water uptake during dry season controls the inter-annual variation in tree growth in four subtropical plantations, Agr. Forest Meteorol., 234–235, 106–114, 2017.
Zhao, L. J., Wang, L. X., Cernusak, L. A., Liu, X. H., Xiao, H. L., Zhou, M. X., and Zhang, S. Q.: Significant difference in hydrogen isotope composition between xylem and tissue water in Populus Euphratica, Plant Cell Environ., 39, 1848–857, 2016.
Zhao, Y. and Wang, L.: Plant water use strategy in response to spatial and temporal variation in precipitation patterns in China: a stable isotope analysis, Forests, 9, 123, https://doi.org/10.3390/f9030123, 2018.
Zhao, Y., Wang, L., Knighton, J., Evaristo, J., and Wassen, M.: Contrasting adaptive strategies by Caragana korshinskii and Salix psammophila in a semiarid revegetated ecosystem, Agr. Forest Meteorol., 300, 108323, https://doi.org/10.1016/j.agrformet.2021.108323, 2021.
Zheng, W. B., Wang, S. Q., Sprenger, M., Liu, B. X., and Cao, J. S.: Response of soil water movement and groundwater recharge to extreme precipitation in a headwater catchment in the North China Plain, J. Hydrol., 576, 466–477, 2019.
Zhu, Y. J. and Shao, M. A.: Variability and pattern of surface moisture on a small-scale hillslope in Liudaogou catchment on the northern Loess Plateau of China, Geoderma, 147, 185–191, 2008.