the Creative Commons Attribution 4.0 License.
the Creative Commons Attribution 4.0 License.
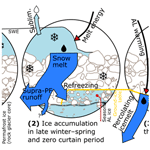
Seasonal ice storage changes and meltwater generation at Murtèl rock glacier (Engadine, eastern Swiss Alps): estimates from measurements and energy budgets in the coarse blocky active layer
Dominik Amschwand
Seraina Tschan
Martin Scherler
Martin Hoelzle
Bernhard Krummenacher
Anna Haberkorn
Christian Kienholz
Lukas Aschwanden
Hansueli Gubler
Intact rock glaciers, a permafrost landform common in high-mountain regions, are often conceptualised as (frozen) water reserves. In a warming climate with slowly degrading permafrost, the large belowground ice volumes might suggest a buffering effect on summer streamflow that due to the climate insensitivity of rock glaciers only increases with rapidly receding glaciers. In this case study, we assess the role and functioning of the intact Murtèl rock glacier in the hydrological cycle of its small (30 ha) periglacial and unglacierised watershed located in the Upper Engadine (eastern Swiss Alps). Our unprecedentedly comprehensive hydro-meteorological measurements include belowground heat flux measurements in the 2–5 m thick coarse blocky active layer (AL), belowground stake measurements of the seasonal evolution of the ground-ice table, and discharge and isotopic signatures of the outflow at the rock-glacier front. The detailed active-layer energy and water/ice balance quantifies precipitation, evaporation, snowmelt, ground-ice melt, and catchment surface outflow. Our single-site, but detailed, case study resolves thermo-hydraulic processes in the coarse blocky AL that might enhance the snowmelt–groundwater connectivity in periglacial high-mountain watersheds underlain by discontinuous permafrost. A substantial part of the snowmelt refreezes in the cold AL (∼ 150–300 mm w.e. or ∼ 20 %–40 % of the snowpack), forming AL ice that is released during the thaw season at melt rates low enough for the meltwater flow to be routed through the permafrost aquitard to deeper sub-permafrost aquifers. Meltwater fluxes are low (1–4 mm w.e. d−1) but sustained throughout the entire thaw season (∼ 100 d) due to small ground heat fluxes and the dampening effect of the AL. The AL ice acts as a coupled thermo-hydrological buffer that (to some extent) protects the underlying ice-rich rock-glacier core by converting most of the ground heat flux to meltwater during the thaw season. Consequently, meltwater release from the old permafrost ice due to climate-induced permafrost degradation is currently ∼ 10 mm yr−1 or an order of magnitude smaller than the contribution of AL meltwater and not more than a few percent of the overall water/ice fluxes. In view of the widespread and long-lasting occurrence of climate-robust permafrost in high-mountain watersheds and the increasing importance of groundwater-sustained late-summer baseflow relative to vanishing glaciers and diminishing snowpacks, it is important to investigate mechanisms, flow paths, and efficiency of groundwater recharge in mountain permafrost terrain.
- Article
(13680 KB) - Full-text XML
- BibTeX
- EndNote
In our rapidly changing climate, changing precipitation patterns and enhanced sublimation/evapotranspiration due to warming and greening (vegetation succession) lead to profound hydrological regime shifts in high-mountain regions (Hock et al., 2022). The hydrological buffer capacity of aboveground cryospheric components decreases as glaciers recede (Hugonnet et al., 2021) and precipitation in the form of snow decreases (Gottlieb and Mankin, 2024), while evaporative losses to the atmosphere (Fugger et al., 2024) and inter-annual variability in precipitation increase. Droughts and reduced streamflow in the summer months of dry years might become more frequent and severe, reducing water security in downstream regions (Haeberli et al., 2017; Schaffer et al., 2019; Hoelzle et al., 2020; Barandun et al., 2020; Arenson et al., 2022). Hence, the hydrological buffer capacity of comparatively climate-resilient and robust belowground components will become increasingly important: ice-rich mountain permafrost, with rock glaciers as its most conspicuous morphological expression (Barsch, 1996), and groundwater in headwater aquifers (Woo, 2011; Hayashi, 2020).
Intact (ice-bearing) rock glaciers, a mountain permafrost landform widespread in nearly all mountain ranges worldwide, are distinct bodies of a perennially frozen debris–ice mixture covered by a seasonally thawed debris layer, the active layer (AL) (Haeberli et al., 2006). The perennially frozen interior, the rock-glacier core, consists of ice-supersaturated debris whose creep deformation results in the conspicuous lobate or tongue-like form (Barsch, 1996). Intact rock glaciers store and release water in different forms (such as ice, snow, and water) on long, intermediate, and short timescales (Jones et al., 2019). The insulating and convective cooling effect of the thick AL creates a cool and stable microclimate in the AL that is partially decoupled from the atmosphere (Wakonigg, 1996; Humlum, 1997; Harris and Pedersen, 1998; Hanson and Hoelzle, 2004; Delaloye and Lambiel, 2005; Guodong et al., 2007; Amschwand et al., 2024, 2025). Controlled by the ground thermal regime rather than by the surface energy balance (SEB) directly (Amschwand et al., 2024), ground-ice melt at depth proceeds slower and is delayed compared to snow or glacier melt at the surface on seasonal up to decadal timescales. On a seasonal timescale, the sustained melt of ground ice is thought to contribute to late-summer streamflow. On decadal timescale, rock glaciers are less sensitive to climate changes compared to (debris-covered) glaciers and are expected to outlast them as our mountains shift away from the glacial towards the paraglacial and periglacial realms (Haeberli et al., 2017, 2024; Schaffer and MacDonell, 2022). Especially in semi-arid, weakly glaciated, and water-stressed high-mountain areas such as parts of Central Asia (Bolch and Marchenko, 2009; Barandun et al., 2020), the Himalayas (Jones et al., 2018b; Harrison et al., 2021; Jeelani et al., 2024), or the Dry Andes (Rangecroft et al., 2015; Janke et al., 2017; Schaffer et al., 2019; Navarro et al., 2023), the large belowground ice volumes and hydrological buffer capacity of climate-resilient, ice-rich permafrost landforms might become important hydrological elements.
The hydrological significance of rock glaciers primarily relates to (1) the climate-resilient storage of permafrost ice in the rock-glacier core (water in reserve), (2) the seasonal storage and freezing/melting of water/ice in the AL (water/ice in circulation), and (3) water storage in unfrozen fine-grained sediments and interaction with liquid water flowing through or beneath rock glaciers (storage–release, routing and chemical alteration/mineralisation of water) (Azócar and Brenning, 2010; Corte, 1976; Burger et al., 1999; Jones et al., 2019). A useful concept for their understanding and management is to differentiate between water/ice in circulation (renewable, flow-limited resource) and water/ice in reserve (nonrenewable, stock-limited resource) based on a timescale of 1 (or a few) hydrological year(s) (Gleick and Palaniappan, 2010). Intact rock glaciers have been storing old ice in the permafrost body beneath the AL for centuries up to millennia (Krainer et al., 2015). This permafrost ice at depth has been interpreted to be roughly as old as the rock glacier it is embedded in. Therefore, it is frozen precipitation from past Holocene cold climatic phases and is a nonrenewable resource (Barsch, 1996; Haeberli et al., 2003; Azócar and Brenning, 2010; Krainer et al., 2015; Amschwand et al., 2021; Lehmann et al., 2022; Nickus et al., 2023). Protected by the AL, build-up and melt processes have been slow and driven by major climatic shifts throughout the Holocene. Driven by the current climate change, permafrost including rock glaciers is found to be degrading widely in high-mountain environments (Beniston et al., 2018; Biskaborn et al., 2019). Intact rock glaciers react by slow AL deepening, releasing meltwater previously bound in the ice-rich permafrost and transitioning towards a relict (ice-free) state over the timescale of centuries (Scherler et al., 2013). As intact rock glaciers are a common periglacial landform and as the ice-rich core is typically 10–30 m thick (Cicoira et al., 2021), the amount of belowground permafrost ice as estimated from rock-glacier inventories and empirical area–volume scaling relations is substantial (Rangecroft et al., 2015; Azócar and Brenning, 2010; Brenning and Azócar, 2010; Brenning, 2005; Jones et al., 2018b, a). In semi-arid and weakly glacierised catchments, water volume equivalent (w.e.) stored in rock glaciers can exceed glacier ice volume or might do so in the future (Jones et al., 2018a; Bodin et al., 2010; Janke et al., 2017; Azócar and Brenning, 2010). Young ice accumulates and melts seasonally in the AL (“superimposed ice” in Bearzot et al., 2023). This ice is derived from modern precipitation and is a renewable resource. Its build-up and release is conditioned by freeze–thaw cycles driven by the SEB and short-term weather conditions. AL ice volumes are presumably much smaller compared to that of permafrost ice but in much faster exchange between the atmosphere and hydrosphere. Available studies suggest that a considerable fraction of the annual precipitation can be stored and released from intact rock glaciers or block fields. Marchenko et al. (2012) report that the observed build-up and melt of 40–60 cm of ground ice seasonally stored a substantial amount (< 30 %) of the snowpack in block fields in the northern Tien Shan. Halla et al. (2021) found inter-annual ice storage changes on Dos Lenguas rock glacier (Dry Andes of Argentina) of −36 mm yr−1 (25 %–80 % of the annual precipitation) and +28 mm yr−1 (17 %–55 %). While the timing of seasonal formation and melt of ground ice has long been inferred from thermal (Hinkel and Outcalt, 1994; Kane et al., 2001; Hanson and Hoelzle, 2003, 2004; Sawada et al., 2003; Rist and Phillips, 2005; Herz, 2006) and geophysical measurements (Hilbich et al., 2009; Schneider et al., 2013), so far few estimates of the magnitude of seasonal to inter-annual ice turnover exist even at the plot scale. These estimates are based on rare exposure of ground ice or drillings (Sawada et al., 2003; Yoshikawa et al., 2023; Marchenko et al., 2012, 2024), and petrophysical joint inversions of (most often) geoelectrical and seismic measurements (Mollaret et al., 2019, 2020; Steiner et al., 2021; Bearzot et al., 2023; Bast et al., 2024), in cases combined with kinematic surveys to relate changes in ground-ice content to surface kinematics (heave/subsidence) (Halla et al., 2021). Quantifying changes in ground-ice content and AL depth is a progressing research field (e.g. Hauck et al., 2011; Wagner et al., 2019; Pavoni et al., 2023; Maierhofer et al., 2024; Morard et al., 2024), because the closer degrading permafrost approaches 0 °C and enters the zero curtain, the more its state is reflected by changes in ice content (latent heat) rather than by ground temperatures (sensible heat) (Hauck and Hilbich, 2024). Other approaches include hydro-chemical and water isotope measurements (Blumstengel and Harris, 1988; Harris et al., 1994; Williams et al., 2006; Munroe and Handwerger, 2023a, b), AL energy budgets (Scherler et al., 2014), and numerical modelling (Pruessner et al., 2022; Renette et al., 2023; Mendoza López et al., 2024). The ice-rich permafrost of intact rock glaciers affects the hydrology of a watershed by its semi-impervious layer (aquitard) that controls the lateral flow and limits (but not prevents) the exchange between surface waters and sub-permafrost groundwater (Cheng and Jin, 2012). A perched supra-permafrost aquifer within the AL on top of the ground-ice table is separated from a sub-permafrost aquifer (and unfrozen water in intra-permafrost taliks). Runoff from the shallow, highly permeable supra-permafrost aquifer is rapid and flashy; this quick flow is only weakly chemically altered. However, some water percolates across the permafrost through taliks or warm permeable zones. These are characteristic features of the inherently discontinuous mountain permafrost, whose distribution is mosaic-like and controlled by spatially complex topo-climatic conditions like insolation/shading and snow cover (Arenson et al., 2022). A dynamic sub-permafrost storage of liquid water retains water in fine-grained, unfrozen sediments for months to years and sustains baseflow during summer droughts or in winter (Rogger et al., 2017; Wagner et al., 2021; Reato et al., 2021; Bearzot et al., 2023). This water is more strongly chemically altered and mineralised, as, for example, indicated by its higher electrical conductivity (EC) (Krainer and Mostler, 2002). For long-term projections, it is important to appreciate the widely diverging timescales of storage mechanisms (from weeks in the AL to millennia in the permafrost core) and the transient state of the mountain permafrost subject to climate change (Jones et al., 2019). As rock glaciers degrade, hydraulic permeability and liquid water storage capacity increase at the expense of the storage of permafrost ice (Winkler et al., 2016a, b; Wagner et al., 2016; Harrington et al., 2018; Colombo et al., 2018).
Although the conceptual framework of the different rock-glacier storage mechanisms is established knowledge, no consensus on the present or future hydrological role of rock glaciers has been reached to date (Duguay et al., 2015; Schaffer et al., 2019; Jones et al., 2019; Arenson et al., 2022), not least due to sparse quantitative hydro-meteorological field data from permafrost-underlain high-mountain watersheds. In this work, we present quantitative data on ground-ice storage changes in the AL of Murtèl rock glacier (Engadine, eastern Swiss Alps) for the hydrological years 2021–2023 based on stake measurements of the seasonally moving ground-ice table and from the AL energy budget. Ground-ice storage changes are energy-controlled phase changes; that is, ice/water and energy turnover in the AL are closely linked. By measuring and parameterising the ground heat fluxes and accounting for sensible heat storage changes in the thick debris mantle, the latent storage changes associated with water phase changes – melting and refreezing – can be isolated. We build on a large body of local previous work: the SEB and AL-internal heat fluxes on Murtèl have been measured/estimated by Mittaz et al. (2000), Hoelzle et al. (2001), Stocker-Mittaz et al. (2002), Schneider (2014), Scherler et al. (2014), Hoelzle et al. (2022), and Amschwand et al. (2024, 2025). Hydro-meteorological measurements (snow, rainfall, outflow discharge) complement the plot-scale water budget. Additionally, we use stable water isotope and electrical conductivity (EC) to compare the rock-glacier outflow to the known δ18O–EC signature of the permafrost ice. This detailed single-site case study contributes to the question of the hydrological significance of rock glaciers by presenting a complete hydro-meteorological data set at the well-studied Murtèl rock glacier, investigates how the permafrost body affects the surface runoff pattern, and explores implications for the permafrost–groundwater connectivity.
2.1 Rock-glacier structure and hydro-morphological setting
The studied Murtèl rock glacier (Murtèl I; WGS 84: 46°25′47′′ N, 9°49′15′′ E; CH1903+/LV95: 2′783′080, 1′144′820; 2620–2700 m a.s.l.; Figs. 1, A1), the close-by Marmugnun rock glacier (Murtèl II), and the relict Murtèl III rock glacier are located in a north-facing cirque in the Upper Engadine, a weakly continental, rain-shadowed high valley in the southeastern Swiss Alps (Fig. 2a). Mean annual air temperature (MAAT) is −1.7 °C, and mean annual precipitation (MAP) is ∼ 900 mm (Scherler et al., 2014). The rock glaciers have an altitude range from 2540 (base of front Murtèl III) or 2620 (base of front Murtèl I) to 2720 m a.s.l. (transition to talus) (Fig. 2b). The talus slopes (at an elevation 2720–2800 m a.s.l.) connect the active rock glaciers to the headwalls and consist of large, angular debris. The headwalls rise from 2800 to 3165 m a.s.l. (a spur of Piz Murtèl) and are more active above the Marmugnun rock glacier (Müller et al., 2014), with massive long-lasting avalanche deposits in late spring to early summer and a debris cone built by frequent rockfalls in summer to autumn. The entire mountain slope is part of the periglacial belt and is underlain by permafrost (Müller et al., 2014). Perennial snow patches/névés reported by Haeberli (1990) and Tenthorey and Gerber (1991) disappeared by the early 2000s (Martin Hoelzle, personal communication, 2021) but were exceptionally present in the cool and wet summer of 2021. Soils are absent and thin, and vegetation is sparse. The catchment is small (30 ha) and not glacierised.
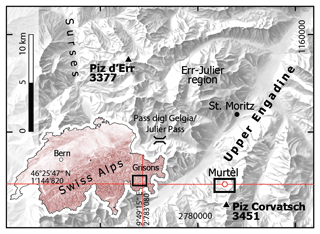
Figure 1Location of Murtèl rock glacier in the Upper Engadine, a high valley in the eastern Swiss Alps. Inset map: location and extent (black rectangle) of the regional map within Switzerland (source: Swiss Federal Office of Topography swisstopo).
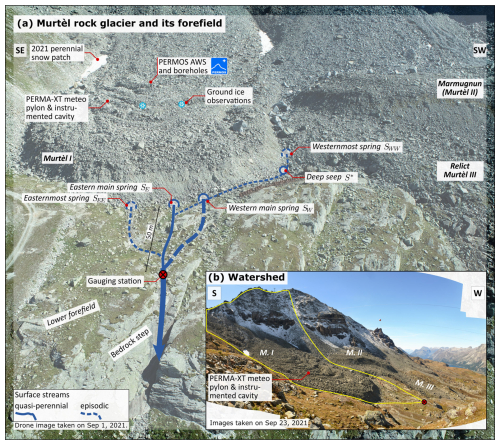
Figure 2(a) Oblique aerial view of the Murtèl rock glacier and its forefield showing location of the rock-glacier springs SEE, SE, SW, SWW, and S∗. Parallel rock ledges show a set of N–S-running fractures. (b) Inset: the Murtèl periglacial catchment.
The lobate Murtèl rock glacier is ca. 300 m long, is 180 m wide, and is covered by a 2–5 m thick coarse blocky AL (debris mantle). Geophysical investigations revealed that its thickness varies according to the surface micro-topography, from 2 m in the furrows to 5 m beneath the ridges (Vonder Mühll and Klingelé, 1994; Vonder Mühll et al., 2000). Thus, the permafrost table shares the surface furrow-and-ridge micro-topography, although attenuated (possible channeling or ponding). Fine material increases towards the AL base but is overall sparse. The permeable coarse blocky AL does not inhibit water flow and has a very low water retention capacity (Springman et al., 2012). The Murtèl permafrost body between the seasonally thawed coarse blocky AL (0–3 m) and bedrock (at 50 m) is comprised of three distinct layers (Vonder Mühll and Haeberli, 1990; Haeberli, 1990; Arenson et al., 2002): (1) massive ice, sparsely bearing sand and silt (3–28 m, supersaturated with over 90 % ice content); (2) a layer of ice-saturated frozen sand (28–32 m) accommodating ca. 60 % of the total/surface displacement (shear horizon); and (3) ice-saturated debris (32–50 m, 40 % ice). The three deep boreholes (Fig. 2), all located within 30 m distance, share this three-part stratigraphy but also reveal lateral small-scale material differences (e.g. laterally variable ice/sand content, lenses) and thermal anomalies (e.g. pointing at non-diffusive heat transfer and intra-permafrost water flow; Vonder Mühll, 1992; Arenson et al., 2010). The extent and ice content of the ice-rich permafrost body is well known from boreholes (Vonder Mühll and Haeberli, 1990; Vonder Mühll, 1992; Vonder Mühll and Holub, 1992; Vonder Mühll and Klingelé, 1994; Haeberli and Vonder Mühll, 1996; Vonder Mühll et al., 2000) and geophysical measurements (electrical resistivity tomography (ERT) and seismic refraction tomography) (Hauck, 2013; Arenson et al., 2010; Mollaret et al., 2019). These data indicate that the ice-rich permafrost core has an extent of 150 × 300 m2 (Christian Hauck, personal communication, 2022), amounting to a water volume equivalent of ∼ 1.5×106 m3. With observations at the surface and during drilling operations, borehole temperature and ERT found evidence for supra- (artesian), intra-, and sub-permafrost water flow (Arenson et al., 2002; Springman et al., 2012). Intra- and sub-permafrost conduits were revealed by water leakage into all drilled boreholes at several depths (Arenson et al., 2002), audible water flow, strong air inflow at the surface, and voids photographed by the borehole camera. Overall, even though Murtèl has a relatively massive and clean ice core compared to other permafrost drill cores (Lazaun; Krainer et al., 2015; Nickus et al., 2023), the permafrost body is far from being impermeable.
The Murtèl rock glacier sits in a bowl-shaped, glacially overdeepened bedrock depression as shown by boreholes and geophysical soundings/gravimetry (Vonder Mühll and Klingelé, 1994). The rock-glacier front has advanced beyond the cirque lip (bedrock sill) onto the forefield that slightly dips away to the north-northwest (NNW). Four rock-glacier springs and one seep emerge between coarse blocks at the base of the rock-glacier front (Fig. 2). The forefield is thinly covered by glacial sediments (till veneer, few large boulders), not perennially frozen (from ERT), and vegetated by grasses (Schneider et al., 2013). There are no surface waters upslope of the rock-glacier springs, and no surface water bodies are impounded in the catchment (except episodically during snowmelt or rainstorms). The bedrock appears fractured. For a few days after strong precipitation, water flows out of bedrock fractures in the steep rock face below the forefield (Fig. 2). The bedrock of the Murtèl cirque predominantly consists of granodiorite, whose blocks make up the bulk of the talus slopes and rock-glacier coarse blocky AL. This Corvatsch granodiorite unit is separated by a tectonic thrust from a westward-thickening seam/wedge of meta-sedimentary units of the Rusenna formation and Blais radiolarite consisting of weakly metamorphosed limestone, mica schists, and radiolarite.
2.2 Selected hydrological and hydro-chemical investigations
We summarise three site-specific past hydrological or water (isotope) chemical studies which are not easily accessible, namely Haeberli (1990), Tenthorey and Gerber (1991), and Stucki (1995).
Murtèl is one of the few rock glaciers where the chemical and isotopic signature of the difficult-to-access permafrost ice, the supposed source of the meltwater, is known from analysed drill cores (Haeberli, 1990). This report provides a concise overview of the isotope (δ2H, δ18O, tritium) and major ion chemistry of the 2/1987 drill core at depths between 3.34 and 20.92 m. The δ18O values of the permafrost ice is in the range of −16 ‰ to −13 ‰, the deuterium excess is in the range of 13 ‰ to 15 ‰, and the δ18O–δ2H relationship is δ2H = 7.97δ18O+12.67 ‰. The electrical conductivity estimated from the major ion concentration is in the range of 5–30 µS cm−1. They interpreted the ground ice as refrozen “diluted groundwater” (non-closed ion balance/cation surplus, pH in the range of 6.3–8.6), likely derived from winter precipitation/snowmelt (syngenetic permafrost formation).
Tenthorey and Gerber (1991) carried out tracer tests in summer 1989 (naphthionate and sodium chloride) and found evidence of two types of water flow: a rapid and channelised flow (supra-permafrost, > 120 m h−1) and a slow and diffuse flow (likely intra-permafrost, < 25 m h−1) (Figs. A1). The Murtèl supra-permafrost water drains predominantly to the main springs SE and SW; a hydrological connection to the deep seep S∗ and even to the relict Murtèl III rock glacier (Fig. 2b) exists but is inefficient. The tracer tests revealed that minor amounts of water leave the catchment via slow groundwater pathways.
Stucki (1995) attempted to establish the provenance of the water that flows in the intra-permafrost bedrock talik at 52–55 m (Sect. 2.1) using stable isotopes as tracers of possible water sources: glacier ice from the nearby Vadret dal Corvatsch, permafrost ice from the same 2/1987 drill core sampled near the ground-ice table, precipitation, and nine springs in the catchment (among them SEE, SE, SW, and SWW). The δ18O of the ground ice was −15.3 ‰, identical to that of the snow sample; the δ18O of the outflow SE became seasonally depleted from −11 ‰ (30 July 1994) to −14 ‰ (7 October) at overall decreasing discharge, and their δ18O–δ2H relationship is δ2H = 8.4δ18O+18.7 ‰ (n = 30, R2 = 0.99).
A comprehensive hydro-meteorological data set is obtained from hydrological sensors (Table 1, Sect. 3.1), from the PERMA-XT and Swiss Permafrost Monitoring Network (PERMOS) automatic weather stations (AWSs) on the rock glacier (Amschwand et al., 2024) (Sect. 3.2), from active-layer sensors complemented with PERMOS borehole temperature data to estimate the AL energy budget (Amschwand et al., 2025) (Sect. 3.3), and direct observation of the ground-ice table (Sect. 3.4). Throughout this publication, the term “meltwater” denotes melt from various types of ground ice but not from snow (explicitly denoted as “snowmelt”) or from glacier ice (the catchment is not glacierised).
Amschwand et al., 2024Amschwand et al., 2025Table 1PERMA-XT sensor specifications. The belowground sensors were operational from September 2020 to September 2023 (destroyed by rockfall).
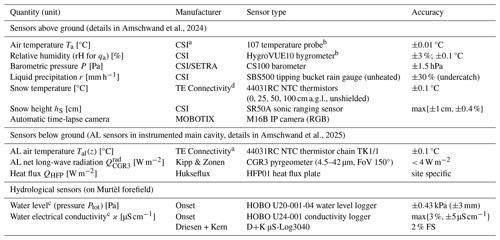
Measurement range and accuracy by manufacturer/vendor. The specifications of the PERMOS sensor are given in Scherler et al. (2014) and Hoelzle et al. (2022).
a Thermistor strings manufactured by Waljag GmbH. b CSI: Campbell Scientific, Inc. Sampling interval: 30 min (or shorter). c All water sensors additionally measure temperature.
FoV represents field of view. FS represents full scale.
3.1 Hydrological measurements
3.1.1 Discharge measurement
The water level gauge is located in the lower forefield after the confluence of all four rock-glacier springs, and it captured the catchment-integrated surface outflow (Fig. 2, Table 1). The discharge Qw is expressed as a power-law relation of water level hw (stage) with empirically fitted coefficients c1 and c2,
where h0 is the stage at zero discharge (standing water in logger pool). The coefficients for the stage–discharge (hw–Qw) relation (Eq. 1) were constrained by dilution gaugings with sodium chloride as a chemical tracer or the volumetric method (bucket method) if discharge was too low for dilution gaugings. The water level is obtained from the total pressure Ptot measured by the submerged logger (pressure compensation) via the hypsometric equation that corrects the barometric pressure measured on the rock glacier to the elevation of the gauging station,
Δz = 42 m is the elevation difference, [K] the layer-averaged virtual temperature (approximated by the actual temperature Ta), the elevation-corrected air pressure, ρw = 103 kg m−3 the water density, g the gravitational acceleration [9.81 m s−2], and R the specific gas constant [287 J kg−1 K−1].
3.1.2 Water electrical conductivity monitoring
The electrical conductivity (EC) of the water was monitored at the two main springs at the rock-glacier front and at the total outflow in the bedrock step downstream of the confluence (Fig. 2). We report water EC referenced to 25 °C, ϰ [µS cm−1], calculated from the measured conductivity ϰϑ [µS cm−1] at water temperature ϑw [°C] via
with a linear temperature compensation factor α = 0.019 °C−1 (Hayashi, 2004; McCleskey et al., 2011). Additionally, we measured EC manually using a WTW LF 320 with a TetraCon 325 probe as a reference for the two quasi-continuously measuring conductivity logger models (Table 1) and when the water loggers were found dry (water level too low).
3.1.3 Stable isotope composition
We took grab samples of the spring snowpack before onset of snowmelt (coring with a plastic tube), the rock-glacier outflow, cumulative rainwater, and supra-permafrost water in a rock-glacier furrow where the ground ice is accessible (at the stake measurement spot; Sect. 3.4, Fig. 2). The water samples were stored in polypropylene bottles with little headspace and tightly sealed with Parafilm in order to minimise evaporation effects.
Water stable isotope composition (δ2H, δ18O) was analysed by cavity ring-down spectroscopy at the Institute of Geology of the University of Bern using a Picarro L2120-i analyser attached to a V1102-i vaporiser.
We report the water stable isotope composition as a δ ratio [‰] of the sample to the Vienna Standard Mean Ocean Water (VSMOW), where δ is the ratio of and . Analytical precision is ±1.0 ‰ for δ2H and ±0.1 ‰ for δ18O. The altitudinal gradient in δ18O does not exceed −0.2 ‰ for every 100 m (annual average) (IAEA/WMO, 2015; Bowen, 2017; Kern et al., 2014). The isotopic differences over the catchment elevation range (2600–3100 m a.s.l.) are within 1 ‰.
3.2 Surface fluxes: precipitation and evaporation
3.2.1 Snow
The PERMA-XT pointwise snow depth measurements hS (sonic ranger data, Table 1) located on a windswept rock-glacier ridge are converted to snow water equivalent (SWE) [kg m−2 = mm w.e.] with the semi-empirical parsimonious Δsnow model (Winkler et al., 2021a). Additionally, to harness the larger footprint of the SEB for a spatially averaged snow depth estimate on the rugged rock-glacier surface, the deviation of the SEB, devSEB, during the snowmelt months is back-calculated to SWE,
where devSEB := (Amschwand et al., 2024). The SEB deviation is the remainder of the turbulent fluxes (QH+QLE) and ground heat flux (QG) subtracted from the surface net radiation (Q∗). Δt refers here to the duration of the snowmelt period as estimated from temperature measurements in the snowpack (0 °C) and using time-lapse imagery from an on-site camera (Table 1).
3.2.2 Rain
Liquid precipitation data are taken from the on-site rain gauge, assuming that precipitation is liquid based on a threshold air temperature of Twb = 2 °C. The rainfall heat flux QPr was estimated via (Sakai et al., 2004; Reid and Brock, 2010)
where Cw = ρwcw [4.18 MJ m−3 K−1] is the water volumetric heat capacity, and r [m3 m−2 s−1] is the rainfall rate intercepted at the surface as measured by our on-site rain gauge or from MeteoSwiss data (Sect. 4.3). Precipitation temperature TP was approximated using the wet-bulb temperature Twb, calculated from air temperature and relative humidity (Amschwand et al., 2024).
3.2.3 Evaporation/sublimation
The evaporative water flux (including sublimation if Ts < 0 °C) is derived from the latent turbulent flux QLE of the Amschwand et al. (2024) SEB that is estimated with the bulk aerodynamic method (Mittaz et al., 2000; Hoelzle et al., 2022). The flux–gradient relation is expressed as
QLE is driven by the specific humidity difference between the atmospheric air qa and the snow (if snow covered) or debris surface (if snow free) qs; qs under snow-free conditions is taken from humidity measurements in the near-surface AL (qa at 0.7 m depth; Table 1). Otherwise, the surface is considered saturated at the radiometrically determined surface temperature. The bulk aerodynamic resistance for vapour transport in the near-surface atmosphere rq [s m−1] decreases with the strength of turbulence: a thermally unstable atmosphere or strong winds enhance turbulent transport. rq is estimated using the Monin–Obukhov similarity theory (MOST) and the parameterisations detailed in Rigon et al. (2006) and Endrizzi et al. (2014).
3.3 AL heat fluxes
3.3.1 The AL energy budget
During the thaw season, the ground heat flux Qg [W m−2] downwards into the coarse blocky AL is spent on warming the debris (sensible heat storage changes), melting ground ice in the AL (latent heat storage change or ground-ice storage change), and conducting into the permafrost body beneath QPF (“permafrost heat flux”) (Woo and Xia, 1996; Hayashi et al., 2007; Boike et al., 2003; Zhu et al., 2024) (Fig. 4),
where ζ is the depth of the ground-ice table (AL thickness) [m], Cv the volumetric heat capacity of the debris [J m−3 K−1], and Tal the AL temperature [°C]. fi [–], Lm [3.35×105 J kg−1], and ρi [kg m−3] are the volumetric ice content, latent heat of melting, and ice density, respectively. The AL energy budget (Eq. 7), derived from the conservation of energy principle, is estimated based on data from the instrumented main cavity (Fig. 2) as outlined below. We compare two independent estimates of the ground-ice melt : one from the deviation of the AL energy budget denoted by deval (Sect. 3.3.3) and one from the Stefan model based on direct stake measurements of ζ(t) in a nearby rock-glacier furrow denoted by Qm (Fig. 2, Sect. 3.4). Details on the measurement set-up and data processing are in Amschwand et al. (2024, 2025).
3.3.2 Ground heat flux Qg
We estimate the thaw-season ground heat flux from two measurements: from the AL net long-wave radiation and the heat flux plate QHFP (Table 1). These two measurements are correlated and represent the downwards heat flux Qg in the absence of buoyancy-driven convection, i.e. in conditions of stably stratified AL air column which prevails during the thaw season. These Qg measurements are at 1.5–2.0 m depth in the AL, not at the surface. Details about data pre-processing are in Amschwand et al. (2025).
3.3.3 Sensible and latent heat storage changes and deval
The sensible heat stored/released by temperature changes of the blocks in the coarse blocky AL beneath the Qg measurement depth is estimated by
where Cv = (1−ϕal)ρrcr is the volumetric heat capacity [0.6 × 2690 kg m−3 × 780 J kg−1 K−1] (porosity ϕal = 0.4), h the distance from the Qg measurement level to the AL base [2 m], and 〈Tal〉 the spatially averaged AL temperatures [°C].
The deviation deval for closure of the AL energy budget (Eq. 7), after assessment of the uncertainties, corresponds to the latent heat storage changes, i.e. heat spent on melting ground ice,
3.3.4 AL base flux through permafrost body QPF
The heat flux across the permafrost table QPF is estimated with the gradient method from PERMOS borehole temperature data via Fourier's heat conduction equation:
where the borehole temperatures are measured at 4 and 5 m depth in the permafrost body beneath the AL. We take a thermal conductivity kPF value of 2.5 W m−1 K−1 (Vonder Mühll and Haeberli, 1990; Scherler et al., 2014).
3.4 Stake measurements at the ground-ice table
3.4.1 Observations of seasonal evolution of the ground-ice table
The ground ice is accessible at a few spots, all located in furrows where the AL is thinner (1–2 m; Fig. 3). In one spot, a plastic tube was drilled ca. 120 cm into the ice in August 2009 but subsequently abandoned (Christin Hilbich, personal communication, 2022). We made serendipitous use of it as an “ablation stake” (Fig. 3b1), manually measuring the depth of the ground-ice table ζ(t) [m] at each field visit in the summers of 2022 and 2023 (Amschwand et al., 2025). Assuming that changes in ice content fi only occur at the phase change boundary ζ(t) coinciding with the 0 °C isotherm (negligible melting point depression in the coarse material), the ice melt heat flux Qm can be expressed as
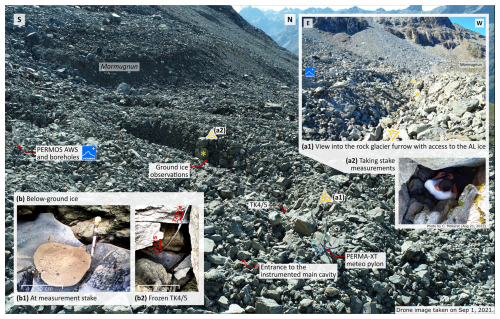
Figure 3Oblique aerial view of the Murtèl rock glacier (foreground) and location of the aboveground sensors and the ground-ice observations in the rock-glacier furrow. Aboveground photographs of (a1) the rock-glacier furrow and (a2) the stake measurements. (b) Belowground ice at (b1) measurement stake and (b2) thermistor TK4/5.
3.4.2 Stefan parameterisation of ground-ice melt
Given the sparse and pointwise stake measurements, we use a Monte Carlo simulation and a Stefan model (Eq. 12) to assess a plausible range of ground-ice melt for a range of input parameter values as expected on the landform scale (probabilistic uncertainty estimate, Sect. 4.4.2). The Stefan model has been widely used to simulate the freezing and thawing fronts in permafrost (e.g. Hayashi et al., 2007; Riseborough et al., 2008; Bonnaventure and Lamoureux, 2013; Hrbáček and Uxa, 2019), including the Cold Regions Hydrological Model (Pomeroy et al., 2022). We parameterise the cumulative heat flux from ground-ice melt on Murtèl = = fiLmρiζ(t) as a function of the depth of the ground-ice table ζ(t) using a modified Stefan equation by Aldrich and Paynter (1953) that is appropriate for a two-layered stratigraphy (Fig. 4) (Kurylyk, 2015; Kurylyk and Hayashi, 2016),
where the surface thawing index I(t) is defined as
and the thaw index of the ice-poor AL overburden I1 is defined as
The factor λ5 ≤ 1 corrects for the sensible heat storage in the thawed layer and is a polynomial of the Stefan number Ste, λ5 = (Kurylyk and Hayashi, 2016). The depth-averaged dimensionless Stefan number Ste is proportional to the ratio of sensible heat to latent heat absorbed during thawing,
with the bulk volumetric heat capacity Cv [J m−3 °C−1] of the (unfrozen, ice-free) AL (identical for both layers), the average surface temperature for the time t elapsed since onset of the thaw season, and the latent heat consumed by the melting of the ground ice Lm〈f〉ρi (different in each layer, and depth-averaged quantities are denoted by 〈⋅〉; details in Kurylyk and Hayashi, 2016).
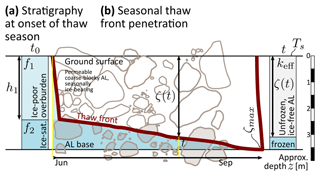
Figure 4Ground-ice thaw and the Stefan equation of a two-layered stratigraphy. (a) Initial stratigraphy at the onset of the thaw season with ice-poor overburden (thickness h1 and ice content f1) and ice-saturated layer (ice content f2 = ϕal). (b) Seasonal thaw front penetration, ζ(t), driven by the ground surface temperature Ts. Maximum thaw depth is denoted by ζmax.
4.1 Meteorological conditions
The weather in each season differed markedly in the 2 years of 2020–2022. Figures 5a and 6a show temperature and precipitation during the summers of 2021 and 2022, respectively. The winter of 2020–2021 was colder than the 2021–2022 one (November–April: average temperature: −6.2 °C vs. −5.3 °C; minimum daily average temperature: −16.5 °C vs. −15.1 °C) and richer in terms of snow amount (November–April: average snow height measured on a windswept ridge: 76 cm vs. 54 cm) and duration (early onset of snow cover: 5 October vs. 3 November; later melt-out: mid-June vs. mid-May). Summer 2021 was cool and wet compared with the hot and dry summer of 2022; temperatures were lower (July–August: average: 6.9 °C vs. 9.3 °C) with frequent passage of synoptic fronts, often bringing cold air (≤ 3 °C; minimum daily average temperature: 0.7 °C vs. 5.6 °C) and mixed precipitation (sleet). Snowfall occurred in a few days throughout the summer and melted within hours. A few snow patches survived over the summer after melt-out of the winter snowpack in mid-June (Fig. 2), which has rarely been occurring in the last ∼ 15 years. A thermistor installed above the ground-ice table in August 2020 became embedded into newly formed ground ice and was only released in August 2022. In contrast, the hot and dry summer of 2022 was marked by three heat waves (in June, July, and August) and daily minimum temperatures not below 5 °C. Several dry spells occurred during this season; the longest one was an 11 d long dry spell within the 5–19 July heat wave. Almost no precipitation was recorded between 20 June and 1 August, despite strong convective precipitation events recorded by the nearby MeteoSwiss station Piz Corvatsch (3294.31 m a.s.l., 1.2 km away). Discharge data of the rock-glacier outflow (Fig. 6b), camera images, and post-event field observations (fresh debris flow deposits, flooding of furrows) revealed rainwater funnelled onto the rock glacier. We augment the PERMA-XT precipitation measurements with MeteoSwiss precipitation data from the station Piz Corvatsch. Immediate on-site inspection of the PERMA-XT rain gauge did not suggest any technical malfunctioning, speaking for a spatially heterogeneous precipitation pattern (Sect. 4.3).
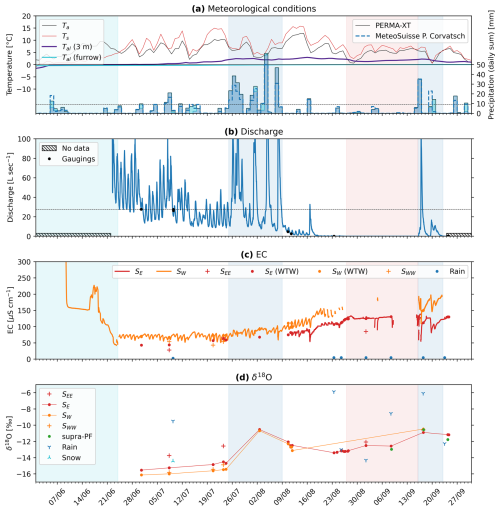
Figure 5Hydro-meteorological data for summer 2021. (a) Temperature and precipitation (daily averages). Thermistor TK4/5 Tal = 0 °C in the furrow remained frozen (location of TK4/5 shown in Fig. 3); 9 mm w.e. d−1 is the infiltration capacity (horizontal dashed line, Fig. 8). (b) Discharge. Maximum gauged discharge is 27.7 L s−1 (horizontal dashed line). (c) Water electrical conductivity (EC). (d) δ18O.
4.2 Hydrological results
Frequent field visits were indispensable to obtain the in situ measurements, to obtain grab samples of water between the coarse blocky material, and to adapt the logger placement to the strongly variable discharge. Suitable places for the EC loggers at the two main springs were found only in late summer of 2021. Still, data gaps due to incompletely submerged loggers at extremely low outflow during hot and dry periods (precisely when meltwater signal can be expected to be clearest) could not be avoided. Six snapshots of the strongly variable discharge in the Murtèl rock-glacier forefield are drawn in Fig. A2. All springs are mentioned in previous investigations (Tenthorey and Gerber, 1991; Stucki, 1995).
4.2.1 Discharge and water temperature
The empirical stage–discharge (hw–Q) relation (Eq. 1) based on eight gaugings (Fig. 7, Table 2) yields the fitted coefficients h0 = 780 mm (stage of the standing water), c1 = 7429, and c2 = 5.15 ± 0.753 (1σ uncertainties; hw in m, Qw in m3 s−1). Because of the wide channel (plane-bed type stream morphology) in the slightly dipping forefield, the water level covered by gaugings varies by merely 9 cm that covers a discharge range from 3 L min−1 (detection threshold) to 27.7 L s−1 (Fig. 7). The channel remained stable during the study period of August 2020–September 2022. Discharge estimates in the wet summer of 2021 rely on extrapolated stage–discharge relation data (often exceeding 27.7 L s−1), while discharge in the dry summer of 2022 is mostly interpolated and better constrained (except the early snowmelt period and peak discharge of event water). Importantly, no stage measurements could be made beneath a snow cover; hence, snowmelt discharge before complete melt-out of the forefield is not gauged. We consider our stage–discharge relation and discharge estimate sufficient for our purpose of season-cumulative water balances and emphasise that our focus is on the low-discharge summer periods where the contribution from ground-ice melt is potentially largest. In the context of the hydrological significance of Murtèl rock glacier, the (reliably measured) zero-discharge estimates will be important.
Discharge in the small catchment is variable and shows a seasonal trend that decreases as snowmelt progresses (Figs. 5, 6), superimposed by regular diurnal fluctuations related to radiative forcing/snowmelt. Total measured discharge was 160×103 m3 in summer 2021 (snowmelt and thaw season) and 97×103 m3 in summer 2022. After completion of the snowmelt, outflow is flashy where dry phases without measurable baseflow (⪅ 3 L min−1) are interrupted by precipitation-fed discharge spikes (event water). The qualitative discharge pattern is similar in both summers. Field observations and additional EC measurements at the bedrock step downstream of the confluence (mixing calculations) suggest that the discharge of SW exceeds that of SE if SW is active. Water temperature of the outflow and the deep seep are stable and always at 0–1 °C.
Table 2Discharge measurements and observations in summer 2021.
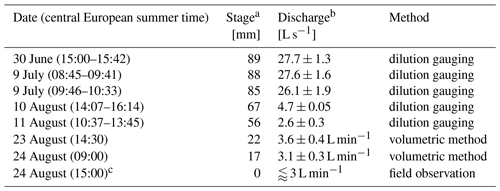
a Stage relative to stage h0. Analytical stage uncertainty of stage measurement: ±3 mm (Table 1). b Analytical discharge uncertainty: standard deviation from three simultaneous EC measurements; 10 % of bucket measurement. c Spring discharge of ⪅ 3 L min−1 seeps away between the spring and the gauging station (detection threshold), and the logger pond runs dry (Fig. A2c).
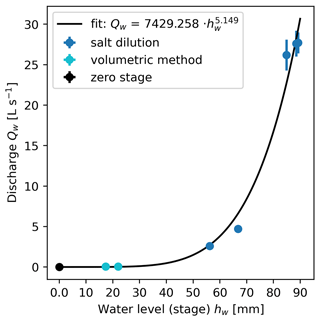
Figure 7Stage–discharge relation as established with dilution gaugings and bucket measurements in summer 2021 (Table 2).
The rainfall–streamflow relation from the threshold analysis (Wagner et al., 2020; Harrington et al., 2018) (Fig. 8) is calculated for the late-summer discharge after completion of the snowmelt in the entire catchment. For our purpose, only one observation is relevant: rainfall less than 9 mm d−1 in most cases (8/10) does not generate measurable outflow at the gauging station (and this finding is independent of the quality of the stage–discharge relation). This agrees with field observations: total spring discharge below the detection threshold of ∼ 3 L min−1 seeps into the ground along its way from the rock-glacier springs to the gauging station located ∼ 50 m below the rock-glacier front (“not measurable baseflow” refers to maximal discharge of ∼ 3 L min−1). We observed rapid recession and drying out of the stilling pool at the gauging station after the discharge estimate in the morning of 24 August 2021 (Fig. A2c, Table 2). We thus constrained the detection limit of ∼ 3 L min−1 using the bucket method (discharge too small for dilution gaugings).
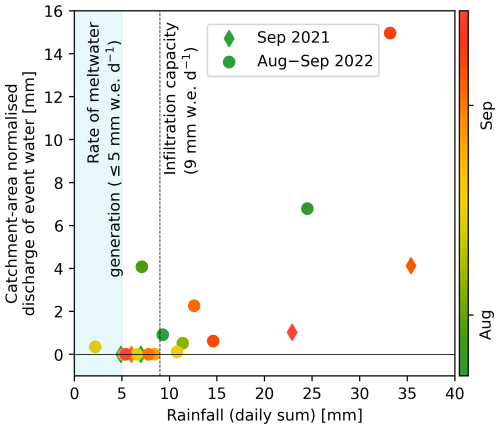
Figure 8Rainfall–streamflow relation (threshold analysis). Rainfall less than 9 mm d−1 did not trigger surface outflow (infiltration capacity). Note that discharge is in cases compounded with discharge from previous rainfalls, introducing some scatter in the rainfall–streamflow relation (e.g. end-July 2022 rainfalls).
4.2.2 Water electrical conductivity
EC of the outflow seasonally increases from ∼ 50 to ∼ 150 µS cm−1 (Figs. 5, 6). Both main springs SE and SW show a qualitatively similar behaviour in both summers, despite different weather conditions: they transition from a snowmelt regime (daily oscillations) to a rain regime (rapid EC drop after onset of event discharge that stabilises). The lower-lying main spring SE (2624 m a.s.l.) has a lower EC than SW (2626 m a.s.l.) and appears more strongly buffered in terms of smaller daily oscillations and smaller seasonal shift. Still, the different weather conditions might show up in the late-season EC: the SE–SW EC difference is largest in autumn 2022 after the dry hydrological year of 2021–2022 (SWE and summer rainfalls below average; Fig. 10), where the EC of SW reaches > 250 µS cm−1. EC is in general anti-correlated with discharge from seasonal down to hourly timescales, suggesting dilution behaviour or longer water residence times: high EC at low discharge which is best seen during snowmelt, although the hourly EC evolution during late-summer rainfalls can be complex with a high-EC peak at the onset of event-water discharge (a timescale beyond the scope of this study). Point measurements of the seep S∗ show a strong enrichment up to 250–350 µS cm−1 of the autumn seep water. The supra-permafrost water co-evolves with the outflow. Notable are the persistent EC difference between two nearby sampling spots in the same rock-glacier furrow: one next to the stake measurement spot and the other next to thermistor TK4/5 (Fig. 2). Spatially varying EC speaks for channelised supra-permafrost flow as interpreted from tracer tests by Tenthorey and Gerber (1991).
4.2.3 Stable isotope signature
We collected a total of 145 samples from different rock-glacier springs and seeps (referred to as outflow), supra-permafrost water, snowpack, and rainwater: 2 samples collected in 2020, 57 samples collected in 2021, and 86 samples collected in 2022 (Table B1). All but a few rainfall samples are aligned on our local meteoric waterline (LMWL) given by ‰ (R2 = 0.9919) (Fig. 9). The δ18O–δ2H relationship of the outflow samples is ‰ (R2 = 0.9969), with its slope of 8.1 similar to the local (LMWL, 8.2) and global meteoric waterline (GMWL, 8.0), suggests that the source waters of the outflow have undergone little if any evaporation. This finding is consistent with a sparsely vegetated coarse blocky landform with rapid infiltration (Williams et al., 2006; Krainer et al., 2007), and it is consistent with the measured specific humidity gradients in the Murtèl AL (Amschwand et al., 2024, 2025): moisture for evaporation is (generally) drawn from a rain-fed reservoir in the shallow AL and not from the supra-permafrost water in the deep AL. Moisture transport in the AL is generally downwards, leading to condensation; upwards transport and evaporation from the deep AL occurs only episodically during droughts.
δ18O of the outflow and supra-permafrost water showed an enrichment during the thaw season from −16 ‰, also measured in the spring snowpack, to −10 ‰, levelling off in late summer to autumn, and repeatedly interrupted by short excursions towards isotopically heavier values of −10 ‰ that co-occur with the isotopically enriched rainfall (typically −10 ‰ to −6 ‰). δ18O was overall higher in summer 2022 and reached the plateau phase sooner (by July), likely reflecting a proportionally smaller amount of snowmelt in the catchment after the snow-poor winter of 2021–2022 (consistent with the EC pattern discussed above). We do not observe the seasonal late-summer isotopic depletion reported by Stucki (1995). The two main springs SE and SW showed, overall, the same pattern, although SW showed seasonally somewhat more extreme values, i.e. isotopically lighter than SE during snowmelt and heavier in late summer. Discrepancies were smallest at high discharge during major rainfall periods. δ18O of the supra-permafrost water was always close to the outflow δ18O. Analogous to its EC, the δ18O value of the supra-permafrost water in the eastern stretch of the furrow is closer to the eastern main spring SE, while the one in the western stretch of the furrow is often closer to spring SW (if active). δ18O values of the rainwater varied considerably between −13 ‰ and −6 ‰ but were generally heavier than all other sampled waters. The denser 2022 data set shows the well-known seasonal pattern with maximum enrichment in July–August. The pattern agreed with 1994–2022 measurements from the nearby Global Network for Isotopes in Precipitation (GNIP) station in Pontresina (1724 m a.s.l.) with ‰ (n = 321, R2 = 0.9943) (accessible via https://nucleus.iaea.org/wiser/, last access: 4 December 2023; IAEA/WMO, 2015). δ18O values of the snowpack were from −15 ‰ to −19 ‰. Although snow δ18O is sensitive to the sampling timing (Beria et al., 2018), its δ18O does not exceed outflow δ18O and is meaningful as a qualitative end-member. The deuterium excess, in cases used as in indicator of multiple freeze–thaw cycles (Williams et al., 2006; Steig et al., 1998; Liaudat Trombotto et al., 2020; Munroe and Handwerger, 2023a, b), shows no clear seasonal trend (Fig. A4).
4.3 Precipitation (snow and rain) and evaporation/sublimation
The plot-scale water balance (Fig. 10) refers to the pointwise (not areal) stake measurements, precipitation measurements, and measurements for the AL energy budget. For the precipitation, we compare different measurements to obtain a spatially representative value (Sect. 4.1):
-
We augment the on-site PERMA-XT rainfall data with MeteoSwiss data from the nearby station Piz Corvatsch. Rainfall data from these stations reasonably agree (Fig. A3) except during a dry window in July 2022 when no on-site precipitation was recorded but rock-glacier outflow occurred whose timing coincides with the MeteoSwiss measurements (Fig. 6a, b). Rain-on-snow events were not considered. The two thaw seasons differed in terms of cumulative precipitation: 460–500 mm in the cool and moist 2021 and ∼ 320 mm in the hot and dry 2022.
-
We use the SEB deviation during the snowmelt period to obtain a representative SWE estimate (“SWE devSEB” in Fig. 10). Comparison with the PERMOS snow height data and time-lapse images shows that the PERMA-XT snow depth measurement located on a windswept ridge grossly underestimates the average SWE on the rugged terrain (at least by a factor of 2.3). Total SWE was 915 mm in the winter of 2020–2021 (average) but only 600 mm in the snow-poor winter of 2021–2022.
The evaporative water flux (Fig. 10) during the thaw season is ≤ 3 mm d−1, amounting to 90 mm in the thaw season of 2021 and 120 mm in 2022. Two remarks are given. First, we consider these values as upper bounds, since the SEB parameterisation in Amschwand et al. (2024) likely tends to overestimate QLE. The additional aerodynamic resistance arising from the vapour transport within the coarse blocky AL is unknown and ignored in Eq. (6). Second, thaw-season vapour transport in the coarse blocky AL is generally downwards, and the specific humidity gradient is aligned with the temperature gradient. It is not the supra-permafrost water that evaporates but meteoric water from the uppermost AL, except during dry spells when the rain-fed moisture store in the shallow AL is exhausted and the specific humidity gradient reverses (Amschwand et al., 2024). In contrast to fine-grained material (or blocky material containing a fine-grained matrix), there is no upwards transport of liquid water by capillary suction (Pérez, 1998).
4.4 Ground-ice storage changes from in situ measurements
4.4.1 Point-scale observations in the AL
The ground ice is rarely accessible in coarse blocky landforms. Here, we present one of the few (to the best of our knowledge) direct observations of the seasonal evolution of AL ice in rock glaciers. The ground-ice table as observed in a rock-glacier furrow in the thaw seasons of 2022–2024 underwent seasonal build-up and melt of ∼ 70 cm within the coarse blocky AL, showing the seasonal build-up and melt of AL ice (Figs. 11, 12). The seasonal release of water from melting ground ice in the coarse blocky AL is 200–300 L m−2 over a thaw period of ∼ 100 d, corresponding to 250 mm water equivalent (w.e.) or a melt rate of 1–4 mm w.e. d−1 (1–4 kg m−2 d−1). A thin (≤ 6 cm) mud layer covered the ground ice in the late summer of 2022. The mud cover loses its insulating effect under the continuous belowground thermal radiation emitted by the blocks (Amschwand et al., 2025; Reznichenko et al., 2010).
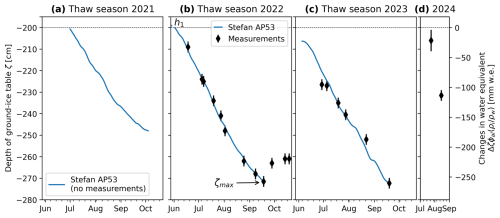
Figure 12Observed and modelled vertical changes in the ground-ice table in the thaw season (a) 2021 (modelled only), (b) 2022, (c) 2023, and (d) 2024 with seasonal build-up and melt. Ice melt is simulated with the Stefan model (Eq. 12).
Our observations indicate two different mechanisms of ground-ice build-up: (1) refreezing of snowmelt onto cold blocks (analogous to the formation of basal ice at the ground–snow interface) and (2) blowing in of snow with subsequent metamorphosis to ice. The “warming spikes”, the AL energy budget, and the ice coatings/icicles (Herz, 2006) in sheltered cavities indicate the former, while the observation of ripe snow on top of the fresh ground ice in exposed cavities indicate the latter mechanism.
4.4.2 Stefan model and probabilistic uncertainty estimate
The Stefan model (Eq. 12) describes the observed lowering of the ground-ice table (“Stefan AP53” in Fig. 12) and relates it to a modelled ground-ice melt Qm. The effective thermal conductivity keff = 3 W m−1 K−1 is derived from the heat flux measurements (Amschwand et al., 2025), the thickness of the ice-poor AL overburden h1 = 3 m, and the ice content f1 = 0.01 is calibrated with the 2022 stake measurements. This 2022 parameter set also describes the 2023 ground-ice melt (Fig. 12c). The ground-ice melt derived from the stake measurements Qm is shown in Fig. 10 (“Ground ice melt AP53”) along with the estimate of the AL energy budget (deval, Sect. 4.5). Systematic stake measurements were not performed in summer 2021. The modelled 2021 ground-ice melt qualitatively agrees with observations insofar as a nearby thermistor (TK4/5) remained embedded in ice in summer 2021 and was only released in July 2022 (Fig. 3b2, Tal in furrow shown in Figs. 5, 6).
Given the sparse and pointwise observations on Murtèl and the few other published observations of seasonal ground-ice melt in coarse blocky landforms, we estimate the uncertainty with a probabilistic Monte Carlo approach. We make an educated guess of the value range of the input parameters. The Stefan melt parameterisation is most sensitive to (Eq. 12), namely the effective thermal conductivity keff (2.0–3.5 W m−1 K−1, Fig. 13c; Amschwand et al., 2025), the AL overburden thickness h1 (1.5–4.0 m, Fig. 13d), and ice content f2 (0.2–0.8, Fig. 13e), assuming that these parameters are independent of each other and of the ground surface temperature Ts. Fifty thousand model runs with the 2021 and 2022 Ts forcing to represent two contrasting thaw seasons yield the outcome distribution of maximum thaw depth ζmax and the total amount of ground-ice melt (Fig. 13b, a). The expected amount of meltwater released in summer 2021 is 100–200 mm w.e. (150–350 mm w.e. in summer 2022), with a large range of overlapping values of 150–250 mm w.e.
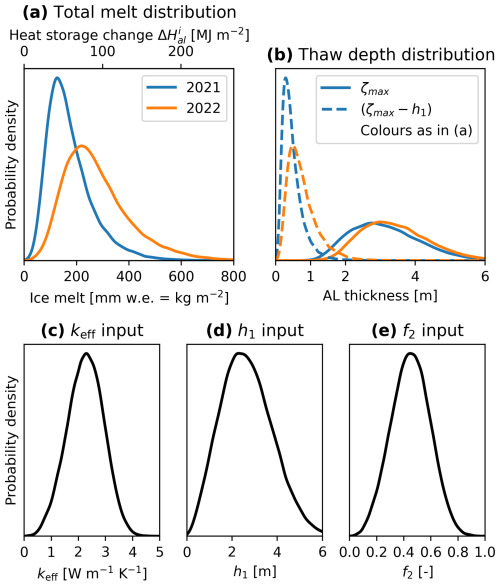
Figure 13Probabilistic uncertainty estimate: distribution of the thaw-season cumulative (a) ground-ice melt and (b) maximum thaw depth ζmax calculated with the 2021 (wet and moist summer) and 2022 (hot and dry summer) Ts forcing (Eq. 12) and a range of AL properties expressed by their input distributions (c) keff, (d) h1, and (e) f2. Variables are the same as in Fig. 4.
4.5 Ground-ice storage changes from the AL energy budget
The ground heat flux estimates were obtained in the instrumented cavity (Fig. 2). Downwards heat flux during the thaw season is typically 10–15 W m−2 as measured by the pyrgeometer QCGR3 and the heat flux plate QHFP (Fig. 14a), amounting to 40–60 MJ m−2 in the cool and wet thaw season of 2021 and 75–95 MJ m−2 in the hot and dry thaw season of 2022 (Fig. 14b, c). The rain heat flux QPr adds another 5–15 MJ m−2. The thaw-season cumulative heat uptake corresponds to less than 10 % of the net surface radiation Q∗ and is spent on warming the coarse blocky AL ( of 10–20 MJ m−2) and transmitted into the permafrost body beneath (QPF of 5–15 MJ m−2). The remaining energy deval of 52–94 MJ m−2 corresponds to a potential ice melt of dev of 160–280 mm w.e. The date of snow melt-out and onset of the thaw season primarily explains the ∼ 40 % larger cumulative heat uptake in 2022 compared to 2021, and the heat uptake scales with the positive degree day (PDD) sum. The direct ground-ice melt estimate Qm from the stake measurements (Fig. 12), here converted to a heat flux via Eq. (11), tends to be larger than deval (“AP53” in Fig. 14), but it agrees well at the end of the thaw season; that is, the estimates of the total ice melt during the thaw season are consistent. Conspicuous warming spikes, rapid AL warming, and downwards heat fluxes occurred in winter–spring and are caused by non-conductive heat transfer. Certainly during the spring snowmelt beneath a thick, closed/insulated snow cover (Amschwand et al., 2024) but possibly also during intra-winter melt events under a thin, discontinuous snow cover, these warming spikes are caused by latent heat effects from refreezing of infiltrated snowmelt rather than air convection (“wind pumping”; Humlum, 1997; Juliussen and Humlum, 2008).
The impact of advective heat transfer by the rainwater increases with depth. In the AL, the warming effect of the rain heat flux is limited compared to the other heat fluxes (radiation, air convection) and overcompensated by the evaporative cooling effect and decreased insolation from the cloud cover during precipitation (Amschwand et al., 2024). Also, the water content in the coarse material remains low and does not affect thermal properties. The effects of drip water on the heat flux plate measurement were not significant for daily to monthly energy budgets as shown by the good correlation to the pyrgeometer measurements (Amschwand et al., 2025). However, in the permafrost body beneath the active layer, heat fluxes are lower, and the effects of rainwater (heat advection, changing thermal and mechanical properties) are not negligible but hard to quantify with our measurements located in the uppermost 3 m.
5.1 Summary of hydro-chemical findings
Many of the hydrological and isotope results on Murtèl rock glacier are along the lines of past studies and are briefly summarised here. A consistent finding is seasonal trends of discharge (decreasing), EC (increasing), and δ18O (increasing) interrupted by precipitation-related excursions (spikes of high discharge, low EC, and heavier δ18O). This pattern is observed in both summers of 2021 and 2022, despite different weather conditions. The synthesis plot EC–δ18O–Q–t (Fig. 15) plots the water samples of the Murtèl outflow as a function of EC, δ18O, discharge Q, and timing t. The plot suggests three end-member components whose contribution varies throughout the summer: (1) snowmelt (depleted isotopic composition, low EC, discharge high for weeks) dominant in early summer, (2) rainwater (enriched δ18O, low EC, discharge episodically high after rainfall) dominant after snowmelt, and (3) groundwater baseflow (“reacted groundwater” of intermediate δ18O, moderate–high EC, very low discharge) to which the system tends to in late summer to autumn (August–October; SE then stagnant with discharge beneath the detection threshold of 3 L min−1). The plot is based on samples from spring SE, which is the last to run dry and provided the most complete data set, extended into autumn 2022 by the then discovered seep Q∗. The other main spring SW is different enough from nearby SE to hint at different water flow paths (a level of detail beyond the scope of this study) yet similar enough to provide a comparable picture. The Y-shape with three end-members based on the three-component model using both chemical (EC) and isotopic (δ18O) tracers agrees with previous studies on intact rock glaciers (Krainer and Mostler, 2002; Harrington et al., 2018; Winkler et al., 2021b). Snowmelt and rock-glacier core/permafrost ice (Haeberli, 1990) have a similar isotopic fingerprint (δ18O of −13 ‰ to −17 ‰). Hence, using isotopes alone, ground-ice meltwater is likely to be indistinguishable from snowmelt. Evidence for ground-ice melt is potentially available during dry phases after snowmelt is completed and when its meltwater largely flushed out of the supra-permafrost aquifer; otherwise, the signal is masked by snowmelt or diluted by rainwater. We suspect that the two strikingly depleted mid-July 2022 supra-permafrost samples collected during a dry spell or heat wave might most closely represent ground-ice melt (−12.9 ‰ and −13.5 ‰, Fig. 6). SE surface outflow at that moment was so low that the carefully placed EC logger was not submerged (data gap); the outflow infiltrated on the spot. Also, the SE outflow was diluted with enriched rainwater, as shown by the δ18O of the manually sampled SE water (−11.4 ‰). The presumed signal of ground-ice melt recorded in the active layer is already lost in the rock-glacier spring, and associated surface outflow is very low (≪ 3 L min−1).
5.2 The Murtèl plot-scale water balance
With our comprehensive hydro-meteorological data set, we resolve the water fluxes and ice storage changes on Murtèl rock glacier on the plot scale for the hydrological years of 2021–2022 (Table 3; Fig. 10), except for groundwater (discussed in Sect. 5.4). The plot scale refers to the pointwise (not areal) stake measurements, precipitation gauging, and measurements for the AL energy budget on the rock glacier. The rock-glacier outflow is normalised by the catchment area (30 ha), inferred from the topography, i.e. the rock glacier and the surrounding talus and rock faces. The thaw-season water budgets are closed, but annual water budgets are not. During the thaw season after near-completion of the snowmelt (July–September 2021 and June–September 2022), surface stream discharge equals available precipitation (precipitation minus evaporation), and there is no sustained baseflow, suggesting that liquid water storage changes are negligible (within the precision of the water budget) and that little rainwater infiltrates (Eq. 1 in Arnoux et al., 2020) (these assumptions are revisited in Sect. 5.4). Discharge during the snowmelt period (April–June 2021 and April–May 2022) and consequently the annual discharge are strongly underestimated, because discharge before snow melt-out of the forefield is not gauged.
The EC and stable isotope signature of the Murtèl surface outflow suggest that there must be some liquid water storage in the catchment which is, however, too small to sustain surface baseflow over more than a few days and is not resolved by the water balance. Evidence for aquifers come from the seasonal increase in EC (a proxy for mineralisation) and the δ18O of the outflow which is buffered to the rainfall δ18O (Figs. 5–6, 15). In particular, the late-summer water sampled in the deep seep S∗ (Fig. 2), which shows a stable δ18O value of −10.9 ± 0.2 ‰ (Fig. 6d), i.e. intermediate between depleted snowmelt (around −15 ‰) and enriched summer rainfall (around −8 ‰), might hint at a sub-permafrost aquifer in the overdeepened bedrock depression (Fig. 16b) (Arenson et al., 2010), recharged from both snowmelt (or ice melt), and rainfall and a water residence time of several months.
Table 3The Murtèl rock-glacier plot-scale water balance in terms of cumulative water fluxes for the respective seasons: winter (October–March), spring snowmelt until the end of the surface zero curtain (May–June/July), thaw-season/summer to autumn (June/July–September), and annual. Values in bold refer to the precipitation data set augmented with MeteoSwiss data from the Piz Corvatsch station (Sect. 4.3).
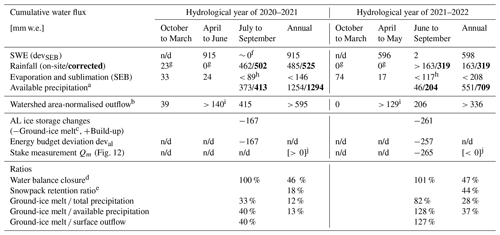
a Available precipitation is rainfall and snowmelt (winter precipitation) minus evaporation/sublimation. b Total surface outflow measured at gauging station (Fig. 2), normalised by catchment area of 30 ha (Fig. 2). c Ground-ice melt is the average of two independent estimates of ground-ice storage changes (Table 3 in Amschwand et al., 2025). d Surface outflow / available precipitation. Deviation arising from infiltration and measurement errors (notably incompletely gauged snowmelt, water level–discharge relation, and uncertain catchment delineation/area). e Amount of melted ground ice (that is derived from the snowpack) divided by the SWE. f Surviving snow patches in catchment (Fig. 2) sustained some snowmelt throughout summer 2021, but it was too small to produce measurable surface outflow. g Rain-on-snow events not quantifiable by our measurement set-up. h Evaporation likely upper bound. i Outflow not gauged when water-level sensor beneath snow cover; no measurable discharge as long as the rock-glacier field is snow covered. j Qualitative observations suggest net build-up in 2020–2021 and net loss in 2021–2022 (Sect. 4.4.2 and Fig. 3b2).
n/d: not determined.
The single largest contribution comes from the snowmelt, which amounts to 65 % of the total annual precipitation released in a few weeks during spring freshet (Table 3; Figs. 5, 6). This is typical for seasonally snow-covered mountain sites. Here, we focus on the contribution of the ground-ice melt compared to precipitation and outflow of the plot-scale water balance (Table 3, Fig. 10). First, in the hot and dry year of 2022 with a snow-poor winter, build-up and melt in coarse blocky AL seasonally stored and released up to 44 % of the winter precipitation (SWE; “snowpack retention ratio” in Table 3) and 28 % of the annual precipitation (37 % of the available precipitation if evaporation/sublimation is subtracted) in the form of temporarily fixed ground ice. In the cool and moist year of 2021 and a winter with average snowfall, the relative importance of the ground-ice melt was smaller: 18 % of SWE and 12 % of the yearly precipitation (13 % of the available precipitation). All these components show a strong, weather-sensitive inter-annual variability. Second, negative ground-ice storage changes amount to 127 % of the surface outflow during the hot and dry summer of 2022 (40 % in the cool, rainy summer 2021), but the meltwater does not appear as surface outflow: the streambed dries out in phases without precipitation (Sect. 5.4). However, even if all of the ice melt reached the surface springs (if the permafrost body were impervious), meltwater exposed to the atmosphere outside of the protecting AL would have a local importance only. Evaporation rates are similarly high as ice melt rates; the meltwater would practically evaporate on the spot (Fig. 10).
5.3 Substantial ground-ice turnover in the AL and little meltwater from degrading permafrost
Most (90 %–100 %) of the generated (ice) meltwater is derived from winter precipitation accumulated in the AL, i.e. from AL thaw not from the ice-rich but quasi-inert permafrost body (rock-glacier core) beneath.
5.3.1 Seasonal ice turnover in the AL
We obtained consistent estimates of seasonal ground-ice melt from the AL energy budget (deval, Fig. 14) and stake measurements (Qm, Figs. 10, 12), although we estimated the AL energy budget deval beneath a broad ridge and made stake measurements Qm in a narrow furrow. End-of-thaw-season values (cumulative ice melt) are 160 mm w.e. in the thaw season of 2021 and 260 mm w.e. in 2022 (Table 3). Although our pointwise observations cannot exclude some differential build-up/melt in furrows and ridges (a micro-topographic variability mentioned by Kääb et al., 1998, and Halla et al., 2021), the agreement suggests that our estimates of end-of-thaw-season ice storage changes are fairly representative over the landform and within an uncertainty of ±50 % (Fig. 13). Smaller discrepancies in the sub-seasonal evolution of Qm and deval (Fig. 14) likely arise from differences in the micro-topographic setting, duration of snow cover, debris texture, and AL thickness at the two measurement points. Our estimates of the ice melt agree with prior studies on Murtèl by Scherler et al. (2014) and Pruessner et al. (2022).
The stake measurements show no local AL thickening for the years 2021–2023 (Fig. 12). The ground ice that melted during the thaw season was regenerated by refreezing and build-up in the following winter and spring. In the exceptionally cool and wet summer of 2021 with late snow melt-out (beginning of thaw season in July), even net build-up occurred at least locally in the rock-glacier furrow (frozen thermistor TK4/5; Fig. 5a). The surviving snow patches (Fig. 2) tentatively support the net positive mass balance observed in 2021 but cannot provide conclusive evidence on the landform scale.
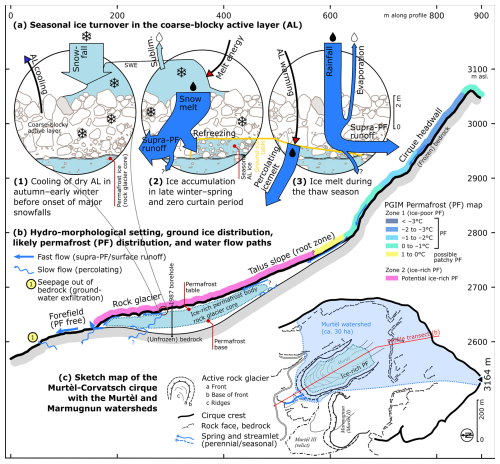
Figure 16Conceptual model of the near-surface hydrogeology of the periglacial Murtèl watershed. (a) Seasonal thermo-hydrological processes in the Murtèl coarse blocky AL. Water fluxes (thick arrows) for the 2021/2022 hydrological year (arrows to scale, Table 3). (b) Profile through the Murtèl watershed. Rock-glacier structure drawn after Vonder Mühll (1993); PGIM represents the permafrost distribution after Kenner et al. (2019). (c) Sketch map of the Murtèl watershed in its cirque.
A net increase in belowground ice content during snowmelt, i.e. the conversion of snowmelt to ground ice, as observed on Murtèl is arguably most efficient on well-drained coarse blocky permafrost landforms. Such terrain, abundant in periglacial high-mountain areas, features a distinct seasonal chain of coupled heat and water–ice transformations co-controlled by the snow cover (illustrated in Fig. 16a: 1–3). In autumn–early winter, the permeable AL contains little water to freeze, enabling a rapid and pervasive ground cooling to large depths before the onset of an insulating snow cover (Renette et al., 2023; Luetschg et al., 2008) (Fig. 16a: 1). In late winter and during spring snowmelt, whenever a warm and melting snowpack releases water into the subfreezing AL, the large cold content (sensible heat) is partly transformed into the build-up of new ground ice (latent heat) (Fig. 16a: 2; warming spikes in Fig. 14). The timing of ground-ice build-up, from snowmelt in spring rather than by freezing soil water in autumn–early winter (Mendoza López et al., 2024), is distinct from fine-grained material with a larger water retention capacity (Renette et al., 2023) and is observed in other coarse blocky permafrost landforms (e.g. Sawada et al., 2003; Rist and Phillips, 2005; Marchenko et al., 2012, 2024). From a hydraulic perspective, the large and permeable pores provide ample storage space for the infiltrating snowmelt to refreeze and for in-blown snow to be trapped at depth without blocking the water infiltration/percolation pathways by the newly formed ground ice (Woo, 2012) or basal ice at the ground–snow interface (Phillips et al., 2017) (but see also Scherler et al., 2010). During the thaw season, the melting AL ice largely (∼ 80 %) absorbs the ground heat flux (Fig. 16a: 3; Amschwand et al., 2025). Numerical simulations by Renette et al. (2023) of the coupled heat and water/ice balance apply to Murtèl. They showed that alone this interplay between the subsurface water/ice balance and ground freezing/thawing leads to negative ground thermal anomalies (up to 2.2 °C in their modelling scenarios), even without air convection which is an additional efficient cooling mechanism common for such terrain (Amschwand et al., 2025; Wicky and Hauck, 2020; Wicky et al., 2024; Amschwand et al., 2025). The regeneration of ground ice in the AL partly explains the climate robustness of coarse blocky landforms (Scherler et al., 2013; Amschwand et al., 2025). If the lost ground ice is not regenerated, the permafrost landform is preconditioned towards irreversible degradation (Hilbich et al., 2008; Hauck and Hilbich, 2024). Finally, permafrost conditions might be required in seasonally snow-covered terrain to keep winter ground temperatures well below the freezing point until the spring snowmelt, depending on the timing and insulation of the snow cover. This is shown by the “bottom temperature of snow cover” (BTS temperatures) (Haeberli, 1973, 1975; Haeberli and Patzelt, 1982), a winter-equilibrium ground surface temperature attained beneath a closed/insulating snow cover that is near or above the freezing point in permafrost-free (seasonally frozen) Alpine terrain. To sum up, ground-ice build-up by snowmelt refreezing relies on strong (preferentially convective) ground cooling and water supply into the subfreezing AL, in turn sensitive to debris texture, thermo-hydraulic properties, and weather/snow conditions.
5.3.2 Melt of permafrost ice in the rock-glacier core
In our warming climate, net build-up as possibly occurred in 2021 is the exception. Murtèl rock glacier is slowly degrading (AL thickening; Noetzli et al., 2019) and must be on a multi-year average releasing meltwater from the old permafrost core. Neither our stake measurements nor our energy balance measurements that span only 2 years can reliably separate melt from young AL ice from melt of old permafrost ice (or a possible transient layer in between; Mendoza López et al., 2024). Inter-annual to decadal surface subsidence estimates from kinematic surveys (Barsch and Hell, 1975; Kääb et al., 1998; Müller et al., 2014, 2016) and vertical borehole deformation (Arenson et al., 2002) revealed slow subsidence attributed to ground-ice melt in the over-saturated permafrost body. Kääb et al. (1998) report 2–5 cm yr−1 for the period 1987–1996. The most recent 2022–2023 geodetic measurements (Isabelle Gärtner-Roer, personal communication, 2024) yield 1 cm yr−1 in the mid–frontal parts of the rock glacier (no significant net change in the surface elevation: the ice melt compensates compressive thickening; subsidence approximately equal to ice loss in massive ice). The amount of meltwater released from the permafrost core is an order of magnitude smaller than the seasonal ice turnover in the AL and negligible compared to precipitation, surface outflow, and even evaporation, agreeing with previous studies (Bearzot et al., 2023).
5.4 Near-surface hydrology of the periglacial Murtèl watershed: meteoric water runs off and ice melt infiltrates
The periglacial Murtèl watershed underlain by either ice-rich permafrost (active rock glacier and talus slope) or steep bedrock (Fig. 16b, c) has a small storage and retention capacity for liquid water (Geiger et al., 2014; Rogger et al., 2017). The thaw-season hydrograph is flashy, responding rapidly (little delay) and strongly (high peak discharge) to daily oscillating snowmelt and rainfall events (Figs. 5, 6). There is no sustained baseflow during winter or summer droughts (⪅ 3 L min−1 for a 30 ha watershed). Once snowmelt is completed, the rock-glacier springs run dry a few days after the last rainfalls. Surface outflow is derived from snowmelt and precipitation (meteoric water, Fig. 16a: 2–3), as shown by the isotopic signature and the thaw-season water balance (Table 3). Importantly, we could neither observe surface runoff from ground-ice melt nor retrieve a clear isotopic signal in the rock-glacier springs during hot and dry weather spells (Figs. 6d, 15b), although extrapolating the melt rates over the ice-underlain rock-glacier area (∼ 150 × 300 m2 à 3 mm w.e. d−1) would yield ∼ 100 L min−1. Whereas snowmelt and rainwater leave the watershed largely as supra-permafrost runoff (some unknown fraction does infiltrate; Fig. 16a: 2–3), no traces of the ice melt are visible (except perhaps on the rock glacier itself, Fig. 15b).
Whereas comparatively intense rainfall that exceeds the infiltration capacity largely runs off as supra-permafrost water and generates surface streamflow, slowly generated ice melt (limited by the small ground heat flux QG) fully infiltrates into the permafrost body. In fact, observed and calculated ground-ice melt rates of 1–4 mm w.e. d−1 (∼ 10−8 m s−1; Fig. 10) are beneath the surface runoff threshold or infiltration capacity of 9 mm d−1 indicated by the rainfall–streamflow relation (Fig. 8). Tracer tests by Tenthorey and Gerber (1991) proved a sub-surface hydraulic connection to the front of the relict Murtèl III rock glacier (Figs. 2, A1). Also, the Murtèl drilling campaigns showed that neither the permafrost body nor the unfrozen bedrock beneath are impermeable: supra-, intra-, and sub-permafrost water flow in conduit-like taliks, water inflow, and air loss during drilling operations are reported in Vonder Mühll and Haeberli (1990), Vonder Mühll (1992), and Arenson et al. (2002, 2010). Halla et al. (2021) estimated that 58 %–89 % of the seasonal meltwater left the Dos Lenguas rock-glacier hydrologic system via groundwater pathways. Cicoira et al. (2019) and Kenner et al. (2020) attribute the widely observed springtime rock-glacier acceleration immediately after onset of the snowmelt to rapid percolation of snowmelt through the permafrost body to the basal shear zone.
The Murtèl periglacial watershed is not a “Teflon basin” (Williams et al., 2015). In mountain permafrost, the ground-ice table (permafrost table) is a semi-impervious aquitard that restricts (but not completely prevents) vertical water flow. Mountain permafrost is inherently discontinuous and characterised by a mosaic distribution of perennially and seasonally cryotic ground with varying ground temperatures and water/ice content that reflects the variable microclimatic conditions in the complex terrain (illustrated in Fig. 16b). Zones of warm permafrost (containing unfrozen water) and taliks enhance the hydraulic permeability (connectivity) of discontinuous permafrost and allow for water movement and groundwater recharge/discharge (Cheng and Jin, 2012; Luethi et al., 2017; Wagner et al., 2020; Arenson et al., 2022; Bearzot et al., 2023). We propose that ice melt in the abundant coarse blocky permafrost landforms – intact rock glaciers, frozen talus slopes, and block fields – contribute to groundwater recharge despite low melt rates, because melt rates are steadily sustained over the entire thaw season (insulating effect of the AL), the infiltration efficiency is high, and the infiltrating ice melt is additional to the infiltrating snowmelt and rainwater (Fig. 16a). As glaciers retreat, permafrost thaws and snowpacks diminish in the rapidly deglacierising mountains, groundwater as the most climatically resilient freshwater resource will be increasingly important to sustain baseflow in the headwaters of rivers originating in mountain ranges (Hayashi, 2020). Additionally, the cold groundwater might sustain icy seeps that are climate refugia for cold-adapted aquatic organisms (Hotaling et al., 2019; Tronstad et al., 2020; Brighenti et al., 2019a, b, 2021; Bearzot et al., 2023). Since coarse blocky permafrost landforms are abundant in periglacial high-mountain areas, understanding their climate-controlled future changes, quantifying subsurface water/ice storage and pathways in mountain permafrost-underlain catchments (Woo, 2011; Louis et al., 2024), and assessing the cryosphere–groundwater connectivity are policy-relevant research topics (van Tiel et al., 2024).
We quantify supra-permafrost ice storage changes, precipitation, evaporation, and snowmelt on the Murtèl rock glacier (Engadine, eastern Swiss Alps) as well as the surface outflow of its periglacial, permafrost-underlain watershed. The single-lobe intact rock glacier (4 ha) and its watershed (30 ha) are small, unglacierised, and sparsely vegetated. Our comprehensive hydro-meteorological measurements resolve the water balance and snow/ice storage changes (melt and build-up) at the plot scale in the coarse blocky active layer (AL) based on precipitation and discharge gaugings, snow height measurements, belowground stake measurements of the ground-ice table, and an AL energy budget derived from in situ heat flux measurements and the surface energy balance (Amschwand et al., 2024).
This case study revealed a substantial seasonal build-up and melt (turnover) of ground ice in the AL and suggests that intact rock glaciers like Murtèl contribute to sustain baseflow by recharging groundwater with meltwater from the seasonal thaw of the ice-rich AL. Since the AL ice is retained winter precipitation, rock glaciers cannot increase total annual runoff or compensate for declining glacier meltwater by means of this AL ice turnover but might play an increasingly important role as climate-robust water-buffering and water-routing landforms in the mountain cryosphere. Specific to permeable and coarse blocky landforms with a small supra-permafrost water retention capacity like Murtèl rock glacier, little water is stored in liquid form. Instead, the water is immobilised by forming ice in the AL: a substantial part of the snowmelt is rapidly fixed as AL ice in winter and spring but slowly released during the thaw season, routing a small but sustained meltwater flow through the low-permeability permafrost aquitard to deeper aquifers.
In numbers, the in situ stake measurements and AL energy budget concurrently suggested substantial seasonal ground-ice turnover in the coarse blocky AL with build-up and melt of 150–300 mm w.e. (160 mm w.e. in 2021, 260 mm w.e. in 2022). So 20 %–40 % of the snowpack is accumulated directly (in-blown snow) or refreezes onto the convectively cooled blocks as snowmelt infiltrates into the coarse blocky AL during winter warm spells and the spring snowmelt, forming annually replenished ground ice. This freeze–thaw storage protracts the snowmelt into late summer and absorbs ∼ 80 % of the thaw-season ground heat flux, effectively constituting a coupled thermo-hydrological buffer that releases meltwater in late summer and protects the underlying permafrost body, contributing to the rock glaciers' climate robustness. Consequently, the amount of meltwater released from the underlying permafrost ice is currently ∼ 10 mm w.e. yr−1, which is an order of magnitude smaller than the AL meltwater contribution and negligible compared to the inter-annual precipitation variability. The meltwater could not be tracked as surface outflow at the rock-glacier springs which run dry a few days after the last precipitation (no surface baseflow after snowmelt). Neither discharge gauging nor hydro-chemical evidence (δ18O natural tracer) hinted at the meltwater. Instead, the Murtèl surface outflow is derived from snowmelt and rainwater. We hypothesise that the generated meltwater percolates deeper and recharges the sub-permafrost groundwater. Ground-ice melt rates are limited by the ground heat flux to 1–4 mm w.e. d−1, which is below the 9 mm d−1 infiltration capacity inferred from the local rainfall–streamflow relation. Sub-surface water pathways have been identified by previous tracer tests (Tenthorey and Gerber, 1991).
While our plot-scale energy and water balance investigations provided detailed and quantitative insights into hydro-thermal processes in the Murtèl AL, integrated hydrogeological studies are needed to address rock glaciers as embedded in their watersheds, namely the connectivity to adjoining landforms and the groundwater, groundwater pathways, and the rock glaciers' capacity to sustain baseflow compared to other high-mountain landforms such as relict rock glaciers, moraines, meadows, and wetlands.
A1 Hydro-morphological sketch map
A2 Field observation: six snapshots of the strongly variable discharge
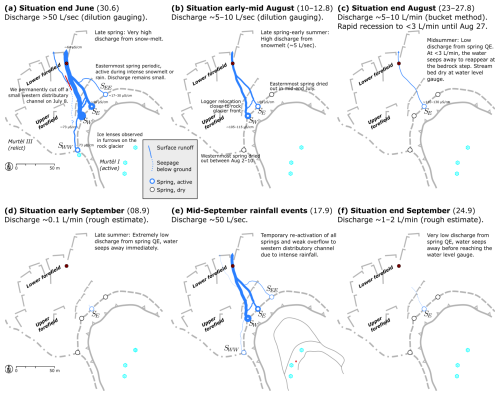
Figure A2Six snapshots of the strongly variable discharge on the rock-glacier forefield in summer 2021 as observed on the regular field visits. (a–c) Spring to early summer (end of June until end of August) with declining discharge as snowmelt progressed. (d–f) Late summer to autumn (end of August until end of September) with strong discharge changes within days as a response to rainfall events. The baseflow in dry periods seeps into the ground before reaching the gauging station in the lower forefield.
A3 Rainfall MeteoSwiss station Piz Corvatsch
Rainfall at the PERMA-XT station on Murtèl and at MeteoSwiss station Piz Corvatsch is shown in Fig. A3.
A4 Deuterium excess dexcess
Deuterium excess [‰] is defined as (Williams et al., 2006)
Results for the thaw seasons of 2021 and 2022 are shown in Fig. A4.
The isotope data are listed in Table B1.
Table B1Water temperature and EC were measured in the field with a WTW probe.
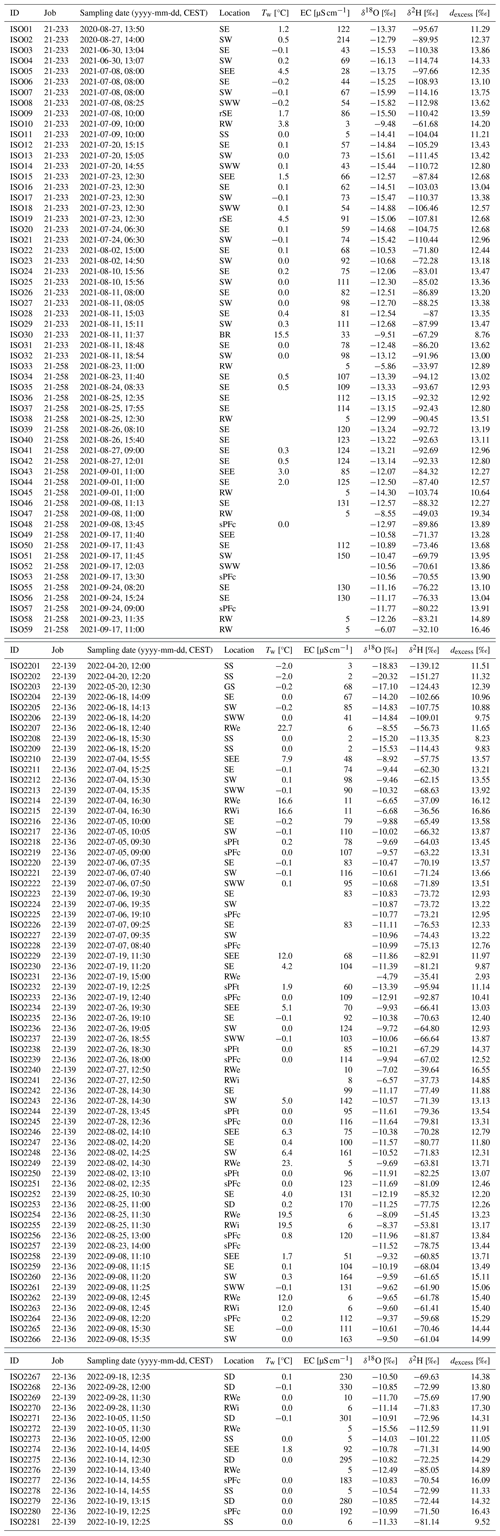
Analytical uncertainty: 0.1 ‰ for δ18O and 1.5 ‰ for δ2H (1σ). See Fig. 2 for sampling locations. Sample locations are abbreviated as follows: GS is stream water at the gauging station. RW is rainwater (RWe is rainwater event, sampled at every field visit; RWi ca. monthly integrated). SS is snow sample. SEE is spring water from the easternmost spring SEE. SE is spring water from the eastern main spring SE. SW is spring water from the western main spring SW. SWW spring water from the westernmost spring SWW. SD is groundwater from the deep seep S∗. rSE is seep in front of relict Murtèl III rock glacier. sPF is supra-permafrost water sampled in rock-glacier furrow (sPFc next to stake measurement, sPFt next to thermistor TK4/5). BR is bedrock seepage (Fig. 2).
The PERMOS data can be obtained from the PERMOS network (https://doi.org/10.13093/permos-meteo-2021-01, Hoelzle et al., 2021) and the PERMA-XT measurement data from https://www.permos.ch/doi/permos-spec-2023-1 (last access: 8 April 2025; https://doi.org/10.13093/permos-spec-2023-01, Amschwand et al., 2023).
DA performed the fieldwork, model development, and analyses for the study and wrote the paper. MS, MH, and BK supervised the study, provided financial and field support, and contributed to the manuscript preparation. AH and CK provided logistical support and editorial suggestions on the manuscript. HG designed the novel sensor array, regularly checked data quality, contributed to the analyses, and provided editorial suggestions on the manuscript.
The contact author has declared that none of the authors has any competing interests.
Publisher’s note: Copernicus Publications remains neutral with regard to jurisdictional claims made in the text, published maps, institutional affiliations, or any other geographical representation in this paper. While Copernicus Publications makes every effort to include appropriate place names, the final responsibility lies with the authors.
This work is a collaboration between the University of Fribourg and GEOTEST. The authors wish to thank Walter Jäger (Waljag GmbH, Malans) and Thomas Sarbach (Sarbach Mechanik, St Niklaus) for the technical support, Stephan Bolay (GEOTEST) for the field assistance (dilution gaugings), and the Corvatsch cable car company for logistical support. Insightful discussions with Theo Jenk (Paul Scherrer Institute PSI), Isabelle Gärtner-Roer and Andreas Vieli (University of Zurich), and Landon Halloran and Clément Roques (University of Neuchâtel) contributed to the paper.
This research has been funded by the Innosuisse – Schweizerische Agentur für Innovationsförderung (grant no. 36242.1 IP-EE, “Permafrost Meltwater Assessment eXpert Tool PERMA-XT”).
This paper was edited by Hongkai Gao and reviewed by Ryan Webb and two anonymous referees.
Aldrich, H. P. and Paynter, H. M.: First Interim Report: Analytical Studies of Freezing and Thawing of Soils, ACFEL Technical Report No. 42, US Corps of Engineers, Boston, MA, http://hdl.handle.net/11681/6526 (last access: 8 April 2025), 1953. a
Amschwand, D., Ivy-Ochs, S., Frehner, M., Steinemann, O., Christl, M., and Vockenhuber, C.: Deciphering the evolution of the Bleis Marscha rock glacier (Val d'Err, eastern Switzerland) with cosmogenic nuclide exposure dating, aerial image correlation, and finite element modeling, The Cryosphere, 15, 2057–2081, https://doi.org/10.5194/tc-15-2057-2021, 2021. a
Amschwand, D., Scherler, M., Hoelzle, M., Krummenacher, B., Tschan, S., Aschwanden, L., and Gubler, H.: Murtèl rock glacier PERMA-XT data set (2020–2023), University of Fribourg/GEOTEST Zollikofen [data set], https://doi.org/10.13093/permos-spec-2023-01, 2023. a
Amschwand, D., Scherler, M., Hoelzle, M., Krummenacher, B., Haberkorn, A., Kienholz, C., and Gubler, H.: Surface heat fluxes at coarse blocky Murtèl rock glacier (Engadine, eastern Swiss Alps), The Cryosphere, 18, 2103–2139, https://doi.org/10.5194/tc-18-2103-2024, 2024. a, b, c, d, e, f, g, h, i, j, k, l, m, n, o
Amschwand, D., Wicky, J., Scherler, M., Hoelzle, M., Krummenacher, B., Haberkorn, A., Kienholz, C., and Gubler, H.: Sub-surface processes and heat fluxes at coarse blocky Murtèl rock glacier (Engadine, eastern Swiss Alps): seasonal ice and convective cooling render rock glaciers climate-robust, Earth Surf. Dynam., 13, 365–401, https://doi.org/10.5194/esurf-13-365-2025, 2025. a, b, c, d, e, f, g, h, i, j, k, l, m, n, o, p
Arenson, L., Hoelzle, M., and Springman, S.: Borehole deformation measurements and internal structure of some rock glaciers in Switzerland, Permafrost and Periglacial Processes, 13, 117–135, https://doi.org/10.1002/ppp.414, 2002. a, b, c, d, e
Arenson, L. U., Hauck, C., Hilbich, C., Seward, L., Yamamoto, Y., and Springman, S. M.: Sub-surface heterogeneities in the Murtèl-Corvatsch rock glacier, Switzerland, in: Proceedings of the joint 63rd Canadian Geotechnical Conference and the 6th Canadian Permafrost Conference (GEO2010), Calgary, Alberta, Canada, 12–15 September 2010, Canadian Geotechnical Society, CNC‐IPA/NRCan, Calgary, AB, Canada, 1494–1500, 2010. a, b, c, d
Arenson, L. U., Harrington, J. S., Koenig, C. E. M., and Wainstein, P. A.: Mountain Permafrost Hydrology – A Practical Review Following Studies from the Andes, Geosciences, 12, 48, https://doi.org/10.3390/geosciences12020048, 2022. a, b, c, d
Arnoux, M., Halloran, L. J. S., Berdat, E., and Hunkeler, D.: Characterizing seasonal groundwater storage in alpine catchments using time-lapse gravimetry, water stable isotopes and water balance methods, Hydrol. Process., 34, 4319–4333, https://doi.org/10.1002/hyp.13884, 2020. a
Azócar, G. F. and Brenning, A.: Hydrological and geomorphological significance of rock glaciers in the dry Andes, Chile (27°–33° S), Permafrost and Periglacial Processes, 21, 42–53, https://doi.org/10.1002/ppp.669, 2010. a, b, c, d
Barandun, M., Fiddes, J., Scherler, M., Mathys, T., Saks, T., Petrakov, D., and Hoelzle, M.: The state and future of the cryosphere in Central Asia, Water Security, 11, 100072, https://doi.org/10.1016/j.wasec.2020.100072, 2020. a, b
Barsch, D.: Rockglaciers. Indicators for the Present and Former Geoecology in High Mountain Environments, in: Springer Series in Physical Environment, Springer Berlin, Heidelberg, Vol. 16, https://doi.org/10.1007/978-3-642-80093-1, 1996. a, b, c
Barsch, D. and Hell, G.: Photogrammetrische Bewegungsmessungen am Blockgletscher Murtèl I, Oberengadin, Schweizer Alpen, Zeitschrift für Gletscherkunde und Glazialgeologie, 11, 111–142, 1975. a
Bast, A., Kenner, R., and Phillips, M.: Short-term cooling, drying, and deceleration of an ice-rich rock glacier, The Cryosphere, 18, 3141–3158, https://doi.org/10.5194/tc-18-3141-2024, 2024. a
Bearzot, F., Colombo, N., Cremonese, E., di Cella, U. M., Drigo, E., Caschetto, M., Basiricò, S., Crosta, G., Frattini, P., Freppaz, M., Pogliotti, P., Salerno, F., Brunier, A., and Rossini, M.: Hydrological, thermal and chemical influence of an intact rock glacier discharge on mountain stream water, Sci. Total Environ., 876, 162777, https://doi.org/10.1016/j.scitotenv.2023.162777, 2023. a, b, c, d, e, f
Beniston, M., Farinotti, D., Stoffel, M., Andreassen, L. M., Coppola, E., Eckert, N., Fantini, A., Giacona, F., Hauck, C., Huss, M., Huwald, H., Lehning, M., López-Moreno, J.-I., Magnusson, J., Marty, C., Morán-Tejéda, E., Morin, S., Naaim, M., Provenzale, A., Rabatel, A., Six, D., Stötter, J., Strasser, U., Terzago, S., and Vincent, C.: The European mountain cryosphere: a review of its current state, trends, and future challenges, The Cryosphere, 12, 759–794, https://doi.org/10.5194/tc-12-759-2018, 2018. a
Beria, H., Larsen, J. R., Ceperley, N. C., Michelon, A., Vennemann, T., and Schaefli, B.: Understanding snow hydrological processes through the lens of stable water isotopes, WIREs Water, 5, e1311, https://doi.org/10.1002/wat2.1311, 2018. a
Biskaborn, B. K., Smith, S. L., Noetzli, J., Matthes, H., Vieira, G., Streletskiy, D. A., Schoeneich, P., Romanovsky, V. E., Lewkowicz, A. G., Abramov, A., Allard, M., Boike, J., Cable, W. L., Christiansen, H. H., Delaloye, R., Diekmann, B., Drozdov, D., Etzelmüller, B., Grosse, G., Guglielmin, M., Ingeman-Nielsen, T., Isaksen, K., Ishikawa, M., Johansson, M., Johannsson, H., Joo, A., Kaverin, D., Kholodov, A., Konstantinov, P., Kröger, T., Lambiel, C., Lanckman, J.-P., Luo, D., Malkova, G., Meiklejohn, I., Moskalenko, N., Oliva, M., Phillips, M., Ramos, M., Sannel, A. B. K., Sergeev, D., Seybold, C., Skryabin, P., Vasiliev, A., Wu, Q., Yoshikawa, K., Zheleznyak, M., and Lantuit, H.: Permafrost is warming at a global scale, Nat. Commun., 10, 264, https://doi.org/10.1038/s41467-018-08240-4, 2019. a
Blumstengel, W. and Harris, S.: Observations on an active lobate rock glacier, Slims River valley, St. Elias Range, Canada, in: Proceedings of the 5th International Conference on Permafrost, Trondheim, Norway, 2–5 August 1988, Tapir Press, Trondheim, 1, 689–695, 1988. a
Bodin, X., Rojas, F., and Brenning, A.: Status and evolution of the cryosphere in the Andes of Santiago (Chile, 33.5° S), Geomorphology, 118, 453–464, https://doi.org/10.1016/j.geomorph.2010.02.016, 2010. a
Boike, J., Roth, K., and Ippisch, O.: Seasonal snow cover on frozen ground: Energy balance calculations of a permafrost site near Ny-Ålesund, Spitsbergen, J. Geophys. Res.-Atmos., 108, ALT 4-1–ALT 4-11, https://doi.org/10.1029/2001JD000939, 2003. a
Bolch, T. and Marchenko, S.: Significance of glaciers, rockglaciers and ice-rich permafrost in the Northern Tien Shan as water towers under climate change conditions, in: Assessment of Snow, Glacier and Water Resources in Asia: Selected papers from the Workshop in Almaty, Kazakhstan, 2006, edited by: Braun, L. N., Hagg, W., Severskiy, I. V., and Young, G., IHP UNESCO, Koblenz, 8, 132–144, 2009. a
Bonnaventure, P. P. and Lamoureux, S. F.: The active layer: A conceptual review of monitoring, modelling techniques and changes in a warming climate, Progress in Physical Geography: Earth and Environment, 37, 352–376, https://doi.org/10.1177/0309133313478314, 2013. a
Bowen, G. J.: The Online Isotopes in Precipitation Calculator, version 3.1, https://wateriso.utah.edu/waterisotopes/pages/data_access/oipc.html (last access: 8 April 2025), 2017. a
Brenning, A.: Geomorphological, hydrological and climatic significance of rock glaciers in the Andes of Central Chile (33–35° S), Permafrost and Periglacial Processes, 16, 231–240, https://doi.org/10.1002/ppp.528, 2005. a
Brenning, A. and Azócar, G. F.: Statistical analysis of topographic and climatic controls and multispectral signatures of rock glaciers in the dry Andes, Chile (27°–33° S), Permafrost and Periglacial Processes, 21, 54–66, https://doi.org/10.1002/ppp.670, 2010. a
Brighenti, S., Tolotti, M., Bruno, M. C., Engel, M., Wharton, G., Cerasino, L., Mair, V., and Bertoldi, W.: After the peak water: the increasing influence of rock glaciers on alpine river systems, Hydrol. Process., 33, 2804–2823, https://doi.org/10.1002/hyp.13533, 2019a. a
Brighenti, S., Tolotti, M., Bruno, M. C., Wharton, G., Pusch, M. T., and Bertoldi, W.: Ecosystem shifts in Alpine streams under glacier retreat and rock glacier thaw: A review, Sci. Total Environ., 675, 542–559, https://doi.org/10.1016/j.scitotenv.2019.04.221, 2019b. a
Brighenti, S., Hotaling, S., Finn, D. S., Fountain, A. G., Hayashi, M., Herbst, D., Saros, J. E., Tronstad, L. M., and Millar, C. I.: Rock glaciers and related cold rocky landforms: Overlooked climate refugia for mountain biodiversity, Glob. Change Biol., 27, 1504–1517, https://doi.org/10.1111/gcb.15510, 2021. a
Burger, K., Degenhardt, J., and Giardino, J.: Engineering geomorphology of rock glaciers, Geomorphology, 31, 93–132, https://doi.org/10.1016/S0169-555X(99)00074-4, 1999. a
Cheng, G. and Jin, H.: Permafrost and groundwater on the Qinghai-Tibet Plateau and in northeast China, Hydrogeol. J., 21, 5–23, https://doi.org/10.1007/s10040-012-0927-2, 2012. a, b
Cicoira, A., Beutel, J., Faillettaz, J., and Vieli, A.: Water controls the seasonal rhythm of rock glacier flow, Earth Planet. Sc. Lett., 528, 115844, https://doi.org/10.1016/j.epsl.2019.115844, 2019. a
Cicoira, A., Marcer, M., Gärtner-Roer, I., Bodin, X., Arenson, L. U., and Vieli, A.: A general theory of rock glacier creep based on in-situ and remote sensing observations, Permafrost and Periglacial Processes, 32, 139–153, https://doi.org/10.1002/ppp.2090, 2021. a
Colombo, N., Salerno, F., Gruber, S., Freppaz, M., Williams, M., Fratianni, S., and Giardino, M.: Review: Impacts of permafrost degradation on inorganic chemistry of surface fresh water, Global Planet. Change, 162, 69–83, https://doi.org/10.1016/j.gloplacha.2017.11.017, 2018. a
Corte, A.: The Hydrological Significance of Rock Glaciers, J. Glaciol., 17, 157–158, https://doi.org/10.3189/S0022143000030859, 1976. a
Delaloye, R. and Lambiel, C.: Evidence of winter ascending air circulation throughout talus slopes and rock glaciers situated in the lower belt of alpine discontinuous permafrost (Swiss Alps), Norsk Geogr. Tidsskr., 59, 194–203, https://doi.org/10.1080/00291950510020673, 2005. a
Duguay, M. A., Edmunds, A., Arenson, L. U., and Wainstein, P. A.: Quantifying the significance of the hydrological contribution of a rock glacier – A review, in: GEOQuébec 2015: Challenges From North to South, Québec, Canada, Canadian Geotechnical Society (CGS), https://www.researchgate.net/publication/282402787 (last access: 8 April 2025), 2015. a
Endrizzi, S., Gruber, S., Dall'Amico, M., and Rigon, R.: GEOtop 2.0: simulating the combined energy and water balance at and below the land surface accounting for soil freezing, snow cover and terrain effects, Geosci. Model Dev., 7, 2831–2857, https://doi.org/10.5194/gmd-7-2831-2014, 2014. a
Fugger, S., Shaw, T. E., Jouberton, A., Miles, E. S., Buri, P., McCarthy, M., Fyffe, C., Fatichi, S., Kneib, M., Molnar, P., and Pellicciotti, F.: Hydrological regimes and evaporative flux partitioning at the climatic ends of high mountain Asia, Environ. Res. Lett., 19, 044057, https://doi.org/10.1088/1748-9326/ad25a0, 2024. a
Geiger, S. T., Daniels, J. M., Miller, S. N., and Nicholas, J. W.: Influence of Rock Glaciers on Stream Hydrology in the La Sal Mountains, Utah, Arct. Antarct. Alp. Res., 46, 645–658, https://doi.org/10.1657/1938-4246-46.3.645, 2014. a
Gleick, P. H. and Palaniappan, M.: Peak water limits to freshwater withdrawal and use, P. Natl. Acad Sci. USA, 107, 11155–11162, https://doi.org/10.1073/pnas.1004812107, 2010. a
Gottlieb, A. R. and Mankin, J. S.: Evidence of human influence on Northern Hemisphere snow loss, Nature, 625, 293–300, https://doi.org/10.1038/s41586-023-06794-y, 2024. a
Guodong, C., Yuanming, L., Zhizhong, S., and Fan, J.: The “thermal semi-conductor” effect of crushed rocks, Permafrost and Periglacial Processes, 18, 151–160, https://doi.org/10.1002/ppp.575, 2007. a
Haeberli, W.: Die Basis-Temperatur der winterlichen Schneedecke als möglicher Indikator für die Verbreitung von Permafrost in den Alpen, Zeitschrift für Gletscherkunde und Glazialgeologie, 9, 221–227, 1973. a
Haeberli, W.: Untersuchungen zur Verbreitung von Permafrost zwischen Flüelapassund Piz Grialetsch (Graubünden), Mitteilungen der VAW/ETH Zürich Nr. 17, VAW/ETH Zürich, https://ethz.ch/content/dam/ethz/special-interest/baug/vaw/vaw-dam/documents/das-institut/mitteilungen/1970-1979/017.pdf (last access: 8 April 2025), 1975. a
Haeberli, W. (Ed.): Pilot analysis of permafrost cores from the active rock glacier Murtèl I, Piz Corvatsch, Eastern Swiss Alps, A workshop report no. 9, Arbeitsheft, VAW/ETH Zürich, 1990. a, b, c, d, e
Haeberli, W. and Patzelt, G.: Permafrostkartierung im Gebiet der Hochebenkar-Blockgletscher, Obergurgl, Ötztaler Alpen, Zeitschrift für Gletscherkunde und Glazialgeologie, 18, 127–150, 1982. a
Haeberli, W. and Vonder Mühll, D.: On the characteristics and possible origins of ice in rock glacier permafrost, Z. Geomorphol. Supp, 104, 43–57, 1996. a
Haeberli, W., Brandova, D., Burga, C., Egli, M., Frauenfelder, R., Kääb, A., Maisch, M., Mauz, B., and Dikau, R.: Methods for absolute and relative age dating of rock-glacier surfaces in alpine permafrost, in: Proceedings of the 8th International Conference on Permafrost, Zurich, Switzerland, 21–25 July 2003, edited by: Phillips, M., Springman, S. M., and Arenson, L. U., Swets & Zeitlinger, Lisse, Zürich, 1, 343–348, iSBN 90 5809 582 7, 2003. a
Haeberli, W., Hallet, B., Arenson, L., Elconin, R., Humlum, O., Kääb, A., Kaufmann, V., Ladanyi, B., Matsuoka, N., Springman, S., and Mühll, D. V.: Permafrost creep and rock glacier dynamics, Permafrost and Periglacial Processes, 17, 189–214, https://doi.org/10.1002/ppp.561, 2006. a
Haeberli, W., Schaub, Y., and Huggel, C.: Increasing risks related to landslides from degrading permafrost into new lakes in de-glaciating mountain ranges, Geomorphology, 293, 405–417, https://doi.org/10.1016/j.geomorph.2016.02.009, 2017. a, b
Haeberli, W., Arenson, L. U., Wee, J., Hauck, C., and Mölg, N.: Discriminating viscous-creep features (rock glaciers) in mountain permafrost from debris-covered glaciers – a commented test at the Gruben and Yerba Loca sites, Swiss Alps and Chilean Andes, The Cryosphere, 18, 1669–1683, https://doi.org/10.5194/tc-18-1669-2024, 2024. a
Halla, C., Blöthe, J. H., Tapia Baldis, C., Trombotto Liaudat, D., Hilbich, C., Hauck, C., and Schrott, L.: Ice content and interannual water storage changes of an active rock glacier in the dry Andes of Argentina, The Cryosphere, 15, 1187–1213, https://doi.org/10.5194/tc-15-1187-2021, 2021. a, b, c, d
Hanson, S. and Hoelzle, M.: The thermal regime of the coarse blocky active layer at the Murtèl rock glacier in the Swiss Alps, in: Proceedings of the 8th International Conference on Permafrost, Zurich, Switzerland, 21–25 July 2003, edited by: Phillips, M., Springman, S. M., and Arenson, L. U., Swets & Zeitlinger, Lisse, Zürich, 51–52, iSBN 90 5809 582 7, 2003. a
Hanson, S. and Hoelzle, M.: The thermal regime of the active layer at the Murtèl rock glacier based on data from 2002, Permafrost and Periglacial Processes, 15, 273–282, https://doi.org/10.1002/ppp.499, 2004. a, b
Harrington, J. S., Mozil, A., Hayashi, M., and Bentley, L. R.: Groundwater flow and storage processes in an inactive rock glacier, Hydrol. Process., 32, 3070–3088, https://doi.org/10.1002/hyp.13248, 2018. a, b, c
Harris, S. A. and Pedersen, D. E.: Thermal regimes beneath coarse blocky materials, Permafrost and Periglacial Processes, 9, 107–120, https://doi.org/10.1002/(SICI)1099-1530(199804/06)9:2<107::AID-PPP277>3.0.CO;2-G, 1998. a
Harris, S. A., Blumstengel, W. K., Cook, D., Krouse, H. R., and Whitley, G.: Comparison of the Water Drainage from an Active Near-Slope Rock Glacier and a Glacier, St. Elias Mountains, Yukon Territory (Vergleich der Entwässerung eines aktiven Hangfuß-Blockgletschers und eines Gletschers, St. Elias Mountains, Yukon-Territorium), Erdkunde, 48, 81–91, http://www.jstor.org/stable/25646552 (last access: 8 April 2025), 1994. a
Harrison, S., Jones, D., Anderson, K., Shannon, S., and Betts, R. A.: Is ice in the Himalayas more resilient to climate change than we thought?, Geogr. Ann. A, 103, 1–7, https://doi.org/10.1080/04353676.2021.1888202, 2021. a
Hauck, C.: New concepts in geophysical surveying and data interpretation for permafrost terrain, Permafrost and Periglacial Processes, 24, 131–137, https://doi.org/10.1002/ppp.1774, 2013. a
Hauck, C. and Hilbich, C.: Preconditioning of mountain permafrost towards degradation detected by electrical resistivity, Environ. Res. Lett., 19, 064010, https://doi.org/10.1088/1748-9326/ad3c55, 2024. a, b
Hauck, C., Böttcher, M., and Maurer, H.: A new model for estimating subsurface ice content based on combined electrical and seismic data sets, The Cryosphere, 5, 453–468, https://doi.org/10.5194/tc-5-453-2011, 2011. a
Hayashi, M.: Temperature-electrical conductivity relation of water for environmental monitoring and geophysical data inversion, Environ. Monit. Assess., 96, 119–128, https://doi.org/10.1023/B:EMAS.0000031719.83065.68, 2004. a
Hayashi, M.: Alpine hydrogeology: The critical role of groundwater in sourcing the headwaters of the world, Groundwater, 58, 498–510, https://doi.org/10.1111/gwat.12965, 2020. a, b
Hayashi, M., Goeller, N., Quinton, W. L., and Wright, N.: A simple heat-conduction method for simulating the frost-table depth in hydrological models, Hydrol. Process., 21, 2610–2622, https://doi.org/10.1002/hyp.6792, 2007. a, b
Herz, T.: Das Mikroklima grobblockiger Schutthalden der alpinen Periglazialstufe und seine Auswirkungen auf Energieaustauschprozesse zwischen Atmosphäre und Lithosphäre [The microclimate of coarse debris covers in the periglacial belt of high mountains and its effects on the energy exchange between atmosphere and lithosphere], PhD thesis, Justus-Liebig-Universität Gießen, Gießen, https://doi.org/10.22029/jlupub-9548, 2006. a, b
Hilbich, C., Hauck, C., Hoelzle, M., Scherler, M., Schudel, L., Völksch, I., Vonder Mühll, D., and Mäusbacher, R.: Monitoring mountain permafrost evolution using electrical resistivity tomography: A 7-year study of seasonal, annual, and long-term variations at Schilthorn, Swiss Alps, J. Geophys. Res.-Earth, 113, F01S90, https://doi.org/10.1029/2007JF000799, 2008. a
Hilbich, C., Marescot, L., Hauck, C., Loke, M. H., and Mäusbacher, R.: Applicability of electrical resistivity tomography monitoring to coarse blocky and ice-rich permafrost landforms, Permafrost and Periglacial Processes, 20, 269–284, https://doi.org/10.1002/ppp.652, 2009. a
Hinkel, K. M. and Outcalt, S. I.: Identification of heat-transfer processes during soil cooling, freezing, and thaw in central alaska, Permafrost and Periglacial Processes, 5, 217–235, https://doi.org/10.1002/ppp.3430050403, 1994. a
Hock, R., Rasul, G., Adler, C., Cáceres, B., Gruber, S., Hirabayashi, Y., Jackson, M., Kääb, A., Kang, S., Kutuzov, S., Milner, A., Molau, U., Morin, S., Orlove, B., and Steltzer, H.: High Mountain Areas, in: IPCC Special Report on the Ocean and Cryosphere in a Changing Climate, edited by: Pörtner, H.-O., Roberts, D. C., Masson-Delmotte, V., Zhai, P., Tignor, M. Poloczanska, E. Mintenbeck, K., Alegrìa, A., Nicolai, M., Okem, A., Petzold, J., Rama, B., and Weyer, N. M., Cambridge University Press, Cambridge, UK and New York, NY, USA, Chap. 4, 131–202, https://doi.org/10.1017/9781009157964.004, 2022. a
Hoelzle, M., Mittaz, C., Etzelmüller, B., and Haeberli, W.: Surface energy fluxes and distribution models of permafrost in European mountain areas: an overview of current developments, Permafrost and Periglacial Processes, 12, 53–68, https://doi.org/10.1002/ppp.385, 2001. a
Hoelzle, M., Barandun, M., Bolch, T., Fiddes, J., Gafurov, A., Muccione, V., Saks, T., and Shahgedanova, M.: The status and role of the alpine cryosphere in Central Asia, in: The Aral Sea Basin: Water for Sustainable Development in Central Asia, edited by: Xenarios, S., Schmidt-Vogt, D., Qadir, M., Janusz-Pawletta, B., and Abdullaev, I., Earthscan Series on Major River Basins of the World, Routledge, London and New York, Chap. 8, 100–121, http://library.oapen.org/handle/20.500.12657/24348 (last access: 8 April 2025), 2020. a
Hoelzle, M., Hauck, C., Mathys, T., Noetzli, J., Pellet, C., and Scherler, M.: Energy balance measurements at three PERMOS sites: Corvatsch, Schilthorn, Stockhorn, PERMOS [data set], https://doi.org/10.13093/permos-meteo-2021-01, 2021. a
Hoelzle, M., Hauck, C., Mathys, T., Noetzli, J., Pellet, C., and Scherler, M.: Long-term energy balance measurements at three different mountain permafrost sites in the Swiss Alps, Earth Syst. Sci. Data, 14, 1531–1547, https://doi.org/10.5194/essd-14-1531-2022, 2022. a, b, c
Hotaling, S., Foley, M. E., Zeglin, L. H., Finn, D. S., Tronstad, L. M., Giersch, J. J., Muhlfeld, C. C., and Weisrock, D. W.: Microbial assemblages reflect environmental heterogeneity in alpine streams, Glob. Change Biol., 25, 2576–2590, https://doi.org/10.1111/gcb.14683, 2019. a
Hrbáček, F. and Uxa, T.: The evolution of a near‐surface ground thermal regime and modeled active‐layer thickness on James Ross Island, Eastern Antarctic Peninsula, in 2006–2016, Permafrost and Periglacial Processes, 31, 141–155, https://doi.org/10.1002/ppp.2018, 2019. a
Hugonnet, R., McNabb, R., Berthier, E., Menounos, B., Nuth, C., Girod, L., Farinotti, D., Huss, M., Dussaillant, I., Brun, F., and Kääb, A.: Accelerated global glacier mass loss in the early twenty-first century, Nature, 592, 726–731, https://doi.org/10.1038/s41586-021-03436-z, 2021. a
Humlum, O.: Active layer thermal regime at three rock glaciers in Greenland, Permafrost and Periglacial Processes, 8, 383–408, https://doi.org/10.1002/(SICI)1099-1530(199710/12)8:4<383::AID-PPP265>3.0.CO;2-V, 1997. a, b
IAEA/WMO: Global Network of Isotopes in Precipitation, The GNIP Database, https://nucleus.iaea.org/wiser (last access: 4 December 2023), 2015. a, b
Janke, J. R., Ng, S., and Bellisario, A.: An inventory and estimate of water stored in firn fields, glaciers, debris-covered glaciers, and rock glaciers in the Aconcagua River Basin, Chile, Geomorphology, 296, 142–152, https://doi.org/10.1016/j.geomorph.2017.09.002, 2017. a, b
Jeelani, G., Hassan, W., Padhya, V., Deshpande, R., Dimri, A., and Lone, S. A.: Significant role of permafrost in regional hydrology of the Upper Indus Basin, India, Sci. Total Environ., 919, 170863, https://doi.org/10.1016/j.scitotenv.2024.170863, 2024. a
Jones, D., Harrison, S., Anderson, K., and Betts, R. A.: Mountain rock glaciers contain globally significant water stores, Nature Scientific Reports, 8, 2834, https://doi.org/10.1038/s41598-018-21244-w, 2018a. a, b
Jones, D., Harrison, S., Anderson, K., Selley, H., Wood, J., and Betts, R.: The distribution and hydrological significance of rock glaciers in the Nepalese Himalaya, Global Planet. Change, 160, 123–142, https://doi.org/10.1016/j.gloplacha.2017.11.005, 2018b. a, b
Jones, D. B., Harrison, S., Anderson, K., and Whalley, W. B.: Rock glaciers and mountain hydrology: A review, Earth-Sci. Rev., 193, 66–90, https://doi.org/10.1016/j.earscirev.2019.04.001, 2019. a, b, c, d
Juliussen, H. and Humlum, O.: Thermal regime of openwork block fields on the mountains Elgåhogna and Sølen, central-eastern Norway, Permafrost and Periglacial Processes, 19, 1–18, https://doi.org/10.1002/ppp.607, 2008. a
Kääb, A., Gudmundsson, G. H., and Hoelzle, M.: Surface deformation of creeping mountain permafrost. Photogrammetric investigations on Murtèl rock glacier, Swiss Alps, in: Proceedings of the 7th International Conference on Permafrost, Yellowknife, Northwest Territories, Canada, 23–27 June 1998, edited by: Lewkowicz, A. G. and Allard, M., Centre d'Études Nordiques, Université Laval (Québec), Canada, 531–537, https://www.arlis.org/docs/vol1/ICOP/40770716/CD-ROM/Proceedings/PDF001189/082308.pdf (last access: 8 April 2025), 1998. a, b, c
Kane, D. L., Hinkel, K. M., Goering, D. J., Hinzman, L. D., and Outcalt, S. I.: Non-conductive heat transfer associated with frozen soils, Global Planet. Change, 29, 275–292, https://doi.org/10.1016/S0921-8181(01)00095-9, 2001. a
Kenner, R., Noetzli, J., Hoelzle, M., Raetzo, H., and Phillips, M.: Distinguishing ice-rich and ice-poor permafrost to map ground temperatures and ground ice occurrence in the Swiss Alps, The Cryosphere, 13, 1925–1941, https://doi.org/10.5194/tc-13-1925-2019, 2019. a
Kenner, R., Pruessner, L., Beutel, J., Limpach, P., and Phillips, M.: How rock glacier hydrology, deformation velocities and ground temperatures interact: Examples from the Swiss Alps, Permafrost and Periglacial Processes, 31, 3–14, https://doi.org/10.1002/ppp.2023, 2020. a
Kern, Z., Kohán, B., and Leuenberger, M.: Precipitation isoscape of high reliefs: interpolation scheme designed and tested for monthly resolved precipitation oxygen isotope records of an Alpine domain, Atmos. Chem. Phys., 14, 1897–1907, https://doi.org/10.5194/acp-14-1897-2014, 2014. a
Krainer, K. and Mostler, W.: Hydrology of Active Rock Glaciers: Examples from the Austrian Alps, Arct. Antarct. Alp. Res., 34, 142–149, https://doi.org/10.1080/15230430.2002.12003478, 2002. a, b
Krainer, K., Mostler, W., and Spötl, C.: Discharge from active rock glaciers, Austrian Alps: a stable isotope approach, Austrian J. Earth Sc., 100, 102–112, https://www.zobodat.at/pdf/MittGeolGes_100_0102-0112.pdf (last access: 8 April 2025), 2007. a
Krainer, K., Bressan, D., Dietre, B., Haas, J. N., Hajdas, I., Lang, K., Mair, V., Nickus, U., Reidl, D., Thies, H., and Tonidandel, D.: A 10,300-year-old permafrost core from the active rock glacier Lazaun, southern Ötztal Alps (South Tyrol, northern Italy), Quaternary Res., 83, 324–335, https://doi.org/10.1016/j.yqres.2014.12.005, 2015. a, b, c
Kurylyk, B. L.: Discussion of 'A simple thaw-freeze algorithm for a multi-layered soil using the Stefan equation' by Xie and Gough (2013), Permafrost and Periglacial Processes, 26, 200–206, https://doi.org/10.1002/ppp.1834, 2015. a
Kurylyk, B. L. and Hayashi, M.: Improved Stefan equation correction factors to accommodate sensible heat storage during soil freezing or thawing, Permafrost and Periglacial Processes, 27, 189–203, https://doi.org/10.1002/ppp.1865, 2016. a, b, c
Lehmann, B., Anderson, R. S., Bodin, X., Cusicanqui, D., Valla, P. G., and Carcaillet, J.: Alpine rock glacier activity over Holocene to modern timescales (western French Alps), Earth Surf. Dynam., 10, 605–633, https://doi.org/10.5194/esurf-10-605-2022, 2022. a
Liaudat Trombotto, D., Sileo, N., and Dapeña, C.: Periglacial water paths within a rock glacier-dominated catchment in the Stepanek area, Central Andes, Mendoza, Argentina, Permafrost and Periglacial Processes, 31, 311–323, https://doi.org/10.1002/ppp.2044, 2020. a
Louis, C., Halloran, L. J. S., and Roques, C.: Seasonal and diurnal freeze-thaw dynamics of a rock glacier and their impacts on mixing and solute transport, EGUsphere [preprint], https://doi.org/10.5194/egusphere-2024-927, 2024. a
Luethi, R., Phillips, M., and Lehning, M.: Estimating non-conductive heat flow leading to intra-permafrost talik formation at the Ritigraben rock glacier (Western Swiss Alps), Permafrost and Periglacial Processes, 28, 183–194, https://doi.org/10.1002/ppp.1911, 2017. a
Luetschg, M., Lehning, M., and Haeberli, W.: A sensitivity study of factors influencing warm/thin permafrost in the Swiss Alps, J. Glaciol., 54, 696–704, https://doi.org/10.3189/002214308786570881, 2008. a
Maierhofer, T., Flores Orozco, A., Roser, N., Limbrock, J. K., Hilbich, C., Moser, C., Kemna, A., Drigo, E., Morra di Cella, U., and Hauck, C.: Spectral induced polarization imaging to monitor seasonal and annual dynamics of frozen ground at a mountain permafrost site in the Italian Alps, The Cryosphere, 18, 3383–3414, https://doi.org/10.5194/tc-18-3383-2024, 2024. a
Marchenko, S., Romanovsky, V., and Gorbunov, A.: Hydrologic and thermal regimes of coarse blocky materials in Tien Shan Mountains, Central Asia, in: Extended abstracts of the 10th International Conference on Permafrost, 25–29 June 2012, Salekhard (Yamal-Nenets Autonomous District), Russia, edited by: Hinkel, K. M. and Melnikov, V. P., Fort Dialog-Iset, Ekaterinburg, Russia, 4, 361–362, 2012. a, b, c
Marchenko, S., Jin, H., Hoelzle, M., Lentschke, J., Kasatkin, N., and Saks, T.: Thermal and hydrologic regimes of blocky materials in Tianshan Mountains, Central Asia, in: Proceedings vol. II (Extendend Abstracts) of the 12th International Conference on Permafrost, Whitehorse (Yukon), Canada, 16–20 June 2024, edited by: Beddoe, R. and Karunaratne, K., International Permafrost Association, 455–456, https://www.permafrost.org/conference-proceedings/ (last access: 8 April 2025), 2024. a, b
McCleskey, R. B., Kirk Nordstrom, D., and Ryan, J. N.: Electrical conductivity method for natural waters, Appl. Geochem., 26, S227–S229, https://doi.org/10.1016/j.apgeochem.2011.03.110, 2011. a
Mendoza López, M., Tapia Baldis, C., Trombotto Liaudat, D., and Pastore, S.: Water content and ground temperature variations in the active layer of a rock glacier in the Central Andes of San Juan, Argentina, Earth Surf. Proc. Land., 49, 3684–3705, https://doi.org/10.1002/esp.5926, 2024. a, b, c
Mittaz, C., Hoelzle, M., and Haeberli, W.: First results and interpretation of energy-flux measurements over Alpine permafrost, Ann. Glaciol., 31, 275–280, https://doi.org/10.3189/172756400781820363, 2000. a, b
Mollaret, C., Hilbich, C., Pellet, C., Flores-Orozco, A., Delaloye, R., and Hauck, C.: Mountain permafrost degradation documented through a network of permanent electrical resistivity tomography sites, The Cryosphere, 13, 2557–2578, https://doi.org/10.5194/tc-13-2557-2019, 2019. a, b
Mollaret, C., Wagner, F. M., Hilbich, C., Scapozza, C., and Hauck, C.: Petrophysical Joint Inversion Applied to Alpine Permafrost Field Sites to Image Subsurface Ice, Water, Air, and Rock Contents, Frontiers in Earth Science, 8, 85, https://doi.org/10.3389/feart.2020.00085, 2020. a
Morard, S., Hilbich, C., Mollaret, C., Pellet, C., and Hauck, C.: 20-year permafrost evolution documented through petrophysical joint inversion, thermal and soil moisture data, Environ. Res. Lett., 19, 074074, https://doi.org/10.1088/1748-9326/ad5571, 2024. a
Müller, J., Vieli, A., and Gärtner-Roer, I.: Rock glaciers on the run – understanding rock glacier landform evolution and recent changes from numerical flow modeling, The Cryosphere, 10, 2865–2886, https://doi.org/10.5194/tc-10-2865-2016, 2016. a
Munroe, J. S. and Handwerger, A. L.: Contribution of rock glacier discharge to late summer and fall streamflow in the Uinta Mountains, Utah, USA, Hydrol. Earth Syst. Sci., 27, 543–557, https://doi.org/10.5194/hess-27-543-2023, 2023a. a, b
Munroe, J. S. and Handwerger, A. L.: Examining the variability of rock glacier meltwater in space and time in high-elevation environments of Utah, United States, Frontiers in Earth Science, 11, 1129314, https://doi.org/10.3389/feart.2023.1129314, 2023b. a, b
Müller, J., Gärtner-Roer, I., Kenner, R., Thee, P., and Morche, D.: Sediment storage and transfer on a periglacial mountain slope (Corvatsch, Switzerland), Geomorphology, 218, 35–44, https://doi.org/10.1016/j.geomorph.2013.12.002, 2014. a, b, c
Navarro, G., MacDonell, S., and Valois, R.: A conceptual hydrological model of semiarid Andean headwater systems in Chile, Progress in Physical Geography: Earth and Environment, 47, 668–686, https://doi.org/10.1177/03091333221147649, 2023. a
Nickus, U., Thies, H., Krainer, K., Lang, K., Mair, V., and Tonidandel, D.: A multi-millennial record of rock glacier ice chemistry (Lazaun, Italy), Frontiers in Earth Science, 11, 1141379, https://doi.org/10.3389/feart.2023.1141379, 2023. a, b
Noetzli, J., Pellet, C., and Staub, B. (Eds.): Permafrost in Switzerland 2014/2015 to 2017/2018, Glaciological Report Permafrost No. 16–19 (PERMOS Report 2019), Cryospheric Commission of the Swiss Academy of Sciences, Fribourg, https://doi.org/10.13093/permos-rep-2019-16-19, 2019. a
Pavoni, M., Boaga, J., Wagner, F., Bast, A., and Phillips, M.: Characterization of rock glaciers environments combining structurally-coupled and petrophysically-coupled joint inversions of electrical resistivity and seismic refraction datasets, J. Appl. Geophys., 215, 105097, https://doi.org/10.1016/j.jappgeo.2023.105097, 2023. a
Phillips, M., Haberkorn, A., and Rhyner, H.: Snowpack characteristics on steep frozen rock slopes, Cold Reg. Sci. Technol., 141, 54–65, https://doi.org/10.1016/j.coldregions.2017.05.010, 2017. a
Pomeroy, J., Brown, T., Fang, X., Shook, K., Pradhananga, D., Armstrong, R., Harder, P., Marsh, C., Costa, D., Krogh, S., Aubry-Wake, C., Annand, H., Lawford, P., He, Z., Kompanizare, M., and Lopez Moreno, J.: The cold regions hydrological modelling platform for hydrological diagnosis and prediction based on process understanding, J. Hydrol., 615, 128711, https://doi.org/10.1016/j.jhydrol.2022.128711, 2022. a
Pruessner, L., Huss, M., and Farinotti, D.: Temperature evolution and runoff contribution of three rock glaciers in Switzerland under future climate forcing, Permafrost and Periglacial Processes, 33, 310–322, https://doi.org/10.1002/ppp.2149, 2022. a, b
Pérez, F. L.: Conservation of soil moisture by different stone covers on alpine talus slopes (Lassen, California), CATENA, 33, 155–177, https://doi.org/10.1016/S0341-8162(98)00091-5, 1998. a
Rangecroft, S., Harrison, S., and Anderson, K.: Rock Glaciers as Water Stores in the Bolivian Andes: An Assessment of Their Hydrological Importance, Arct. Antarct. Alp. Res., 47, 89–98, https://doi.org/10.1657/AAAR0014-029, 2015. a, b
Reato, A., Silvina Carol, E., Cottescu, A., and Alfredo Martínez, O.: Hydrological significance of rock glaciers and other periglacial landforms as sustenance of wet meadows in the Patagonian Andes, J. S. Am. Earth Sc., 111, 103471, https://doi.org/10.1016/j.jsames.2021.103471, 2021. a
Reid, T. D. and Brock, B. W.: An energy-balance model for debris-covered glaciers including heat conduction through the debris layer, J. Glaciology, 56, 903–916, https://doi.org/10.3189/002214310794457218, 2010. a
Renette, C., Aalstad, K., Aga, J., Zweigel, R. B., Etzelmüller, B., Lilleøren, K. S., Isaksen, K., and Westermann, S.: Simulating the effect of subsurface drainage on the thermal regime and ground ice in blocky terrain in Norway, Earth Surf. Dynam., 11, 33–50, https://doi.org/10.5194/esurf-11-33-2023, 2023. a, b, c, d
Reznichenko, N., Davies, T., Shulmeister, J., and McSaveney, M.: Effects of debris on ice-surface melting rates: an experimental study, J. Glaciol., 56, 384–394, https://doi.org/10.3189/002214310792447725, 2010. a
Rigon, R., Bertoldi, G., and Over, T. M.: GEOtop: a distributed hydrological model with coupled water and energy budgets, J. Hydrometeorol., 7, 371–388, https://doi.org/10.1175/JHM497.1, 2006. a
Riseborough, D., Shiklomanov, N., Etzelmüller, B., Gruber, S., and Marchenko, S.: Recent advances in permafrost modelling, Permafrost and Periglacial Processes, 19, 137–156, https://doi.org/10.1002/ppp.615, 2008. a
Rist, A. and Phillips, M.: First results of investigations on hydrothermal processes within the active layer above alpine permafrost in steep terrain, Norsk Geogr. Tidsskr., 59, 177–183, https://doi.org/10.1080/00291950510020574, 2005. a, b
Rogger, M., Chirico, G. B., Hausmann, H., Krainer, K., Brückl, E., Stadler, P., and Blöschl, G.: Impact of mountain permafrost on flow path and runoff response in a high alpine catchment, Water Resour. Res., 53, 1288–1308, https://doi.org/10.1002/2016WR019341, 2017. a, b
Sakai, A., Fujita, K., and Kubota, J.: Evaporation and percolation effect on melting at debris-covered Lirung Glacier, Nepal Himalayas, 1996, Bulletin of Glaciological Research, 21, 9–15, 2004. a
Sawada, Y., Ishikawa, M., and Ono, Y.: Thermal regime of sporadic permafrost in a block slope on Mt. Nishi-Nupukaushinupuri, Hokkaido Island, Northern Japan, Geomorphology, 52, 121–130, https://doi.org/10.1016/S0169-555X(02)00252-0, 2003. a, b, c
Schaffer, N. and MacDonell, S.: Brief communication: A framework to classify glaciers for water resource evaluation and management in the Southern Andes, The Cryosphere, 16, 1779–1791, https://doi.org/10.5194/tc-16-1779-2022, 2022. a
Schaffer, N., MacDonell, S., Réveillet, M., Yáñez, E., and Valois, R.: Rock glaciers as a water resource in a changing climate in the semiarid Chilean Andes, Reg. Environ. Change, 19, 1263–1279, https://doi.org/10.1007/s10113-018-01459-3, 2019. a, b, c
Scherler, M., Hauck, C., Hoelzle, M., Stähli, M., and Völksch, I.: Meltwater infiltration into the frozen active layer at an alpine permafrost site, Permafrost and Periglacial Processes, 21, 325–334, https://doi.org/10.1002/ppp.694, 2010. a
Scherler, M., Hauck, C., Hoelzle, M., and Salzmann, N.: Modeled sensitivity of two alpine permafrost sites to RCM-based climate scenarios, J. Geophysical Res.-Earth, 118, 780–794, https://doi.org/10.1002/jgrf.20069, 2013. a, b
Scherler, M., Schneider, S., Hoelzle, M., and Hauck, C.: A two-sided approach to estimate heat transfer processes within the active layer of the Murtèl–Corvatsch rock glacier, Earth Surf. Dynam., 2, 141–154, https://doi.org/10.5194/esurf-2-141-2014, 2014. a, b, c, d, e, f
Schneider, S.: The heterogeneity of mountain permafrost – A field-based analysis of different periglacial materials, PhD thesis, University of Fribourg, Switzerland, 184 pp., 2014. a
Schneider, S., Daengeli, S., Hauck, C., and Hoelzle, M.: A spatial and temporal analysis of different periglacial materials by using geoelectrical, seismic and borehole temperature data at Murtèl–Corvatsch, Upper Engadin, Swiss Alps, Geogr. Helv., 68, 265–280, https://doi.org/10.5194/gh-68-265-2013, 2013. a, b
Springman, S. M., Arenson, L. U., Yamamoto, Y., Maurer, H., Kos, A., Buchli, T., and Derungs, G.: Multidisciplinary investigations on three rock glaciers in the swiss alps: legacies and future perspectives, Geogr. Ann. A, 94, 215–243, https://doi.org/10.1111/j.1468-0459.2012.00464.x, 2012. a, b
Steig, E. J., Fitzpatrick, J. J., Noel Potter, j., and Clark, D. H.: The geochemical record in rock glaciers, Geogr. Ann. A, 80, 277–286, https://doi.org/10.1111/j.0435-3676.1998.00043.x, 1998. a
Steiner, M., Wagner, F. M., Maierhofer, T., Schöner, W., and Flores Orozco, A.: Improved estimation of ice and water contents in alpine permafrost through constrained petrophysical joint inversion: The Hoher Sonnblick case study, GEOPHYSICS, 86, WB61–WB75, https://doi.org/10.1190/geo2020-0592.1, 2021. a
Stocker-Mittaz, C., Hoelzle, M., and Haeberli, W.: Modelling alpine permafrost distribution based on energy-balance data: a first step, Permafrost and Periglacial Processes, 13, 271–282, https://doi.org/10.1002/ppp.426, 2002. a
Stucki, T.: Permafrosttemperaturen im Oberengadin. Eine Auswertung der Bohrlochtemperaturen im alpinen Permafrost des Oberengadins im Hinblick auf einen Erwärmungstrend und Schmelzwasserabfluss aus dem Permafrost, Master's thesis, Abteilung Erdwissenschaften der ETH Zürich, 1995. a, b, c, d
Tenthorey, G. and Gerber, E.: Hydrologie du glacier rocheux de Murtèl (Grisons). Description et interprétation de traçages d'eau, in: Modèles en Géomorphologie – exemples Suisses. Session scientfique de la Société suisse de Géomorphologie, Fribourg, 22/23 juin 1990, edited by: Monbaron, M. and Haeberli, W., Rapport et recherches, Institut de Géographie Fribourg, Vol. 3, 1991. a, b, c, d, e, f, g
Tronstad, L. M., Hotaling, S., Giersch, J. J., Wilmot, O. J., and Finn, D. S.: Headwaters fed by subterranean ice: Potential climate refugia for mountain stream communities?, West. N. Am. Naturalist, 80, 395–407, https://doi.org/10.3398/064.080.0311, 2020. a
van Tiel, M., Aubry-Wake, C., Somers, L., Andermann, C., Avanzi, F., Baraer, M., Chiogna, G., Daigre, C., Das, S., Drenkhan, F., Farinotti, D., Fyffe, C. L., de Graaf, I., Hanus, S., Immerzeel, W., Koch, F., McKenzie, J. M., Müller, T., Popp, A. L., Saidaliyeva, Z., Schaefli, B., Schilling, O. S., Teagai, K., Thornton, J. M., and Yapiyev, V.: Cryosphere–groundwater connectivity is a missing link in the mountain water cycle, Nature Water, 2, 624–637, https://doi.org/10.1038/s44221-024-00277-8, 2024. a
Vonder Mühll, D. and Haeberli, W.: Thermal characteristics of the permafrost within an active rock glacier (Murtèl/Corvatsch, Grisons, Swiss Alps), J. Glaciol., 36, 151–158, https://doi.org/10.3189/S0022143000009382, 1990. a, b, c, d
Vonder Mühll, D. S.: Evidence of intrapermafrost groundwater flow beneath an active rock glacier in the Swiss Alps, Permafrost and Periglacial Processes, 3, 169–173, https://doi.org/10.1002/ppp.3430030216, 1992. a, b, c
Vonder Mühll, D. S.: Geophysikalische Untersuchungen im Permafrost des Oberengadins, PhD thesis, Versuchsanstalt für Wasserbau, Hydrologie und Glaziologie (VAW), ETH Zürich, https://doi.org/10.3929/ethz-a-000891391, 1993. a
Vonder Mühll, D. S. and Holub, P.: Borehole logging in alpine permafrost, upper Engadin, Swiss Alps, Permafrost and Periglacial Processes, 3, 125–132, https://doi.org/10.1002/ppp.3430030209, 1992. a
Vonder Mühll, D. S. and Klingelé, E. E.: Gravimetrical investigation of ice-rich permafrost within the rock glacier Murtèl-Corvatsch (upper Engadin, Swiss Alps), Permafrost and Periglacial Processes, 5, 13–24, https://doi.org/10.1002/ppp.3430050103, 1994. a, b, c
Vonder Mühll, D. S., Hauck, C., and Lehmann, F.: Verification of geophysical models in Alpine permafrost using borehole information, Ann. Glaciol., 31, 300–306, https://doi.org/10.3189/172756400781820057, 2000. a, b
Wagner, T., Pauritsch, M., and Winkler, G.: Impact of relict rock glaciers on spring and stream flow of alpine watersheds: Examples of the Niedere Tauern Range, Eastern Alps (Austria), Austrian J. Earth Sc., 109, 84–98, https://doi.org/10.17738/ajes.2016.0006, 2016. a
Wagner, T., Pauritsch, M., Mayaud, C., Kellerer-Pirklbauer, A., Thalheim, F., and Winkler, G.: Controlling factors of microclimate in blocky surface layers of two nearby relict rock glaciers (Niedere Tauern Range, Austria), Geogr. Ann. A, 101, 310–333, https://doi.org/10.1080/04353676.2019.1670950, 2019. a
Wagner, T., Brodacz, A., Krainer, K., and Winkler, G.: Active rock glaciers as shallow groundwater reservoirs, Austrian Alps, Grundwasser – Zeitschrift der Fachsektion Hydrogeologie, 25, 215–230, https://doi.org/10.1007/s00767-020-00455-x, 2020. a, b
Wagner, T., Kainz, S., Helfricht, K., Fischer, A., Avian, M., Krainer, K., and Winkler, G.: Assessment of liquid and solid water storage in rock glaciers versus glacier ice in the Austrian Alps, Sci. Total Environ., 800, 149593, https://doi.org/10.1016/j.scitotenv.2021.149593, 2021. a
Wakonigg, H.: Unterkühlte Schutthalden [Undercooled talus], Arbeiten aus dem Institut für Geographie der Karl-Franzens Universität Graz, 33, 209–223, https://www.zobodat.at/pdf/Arb-Inst-Geographie-Uni-Graz_33_1996_0209-0223.pdf (last access: 8 April 2025), 1996. a
Wicky, J. and Hauck, C.: Air convection in the active layer of rock glaciers, Frontiers in Earth Science, 8, 335, https://doi.org/10.3389/feart.2020.00335, 2020. a
Wicky, J., Hilbich, C., Delaloye, R., and Hauck, C.: Modeling the link between air convection and the occurrence of short‐term permafrost in a low‐altitude cold talus slope, Permafrost and Periglacial Processes, 35, 202–217, https://doi.org/10.1002/ppp.2224, 2024. a
Williams, M. W., Knauf, M., Caine, N., Liu, F., and Verplanck, P. L.: Geochemistry and source waters of rock glacier outflow, Colorado Front Range, Permafrost and Periglacial Processes, 17, 13–33, https://doi.org/10.1002/ppp.535, 2006. a, b, c, d
Williams, M. W., Hood, E., Molotch, N. P., Caine, N., Cowie, R., and Liu, F.: The 'teflon basin' myth: hydrology and hydrochemistry of a seasonally snow-covered catchment, Plant Ecol. Divers., 8, 639–661, https://doi.org/10.1080/17550874.2015.1123318, 2015. a
Winkler, G., Pauritsch, M., Wagner, T., and Kellerer-Pirklbauer, A.: Reliktische Blockgletscher als Grundwasserspeicher in Alpinen Einzugsgebieten der Niederen Tauern, Berichte der Wasserwirtschaftlichen Planung Steiermark 87, https://www.wasserwirtschaft.steiermark.at/cms/dokumente/11913323_102332494/6885027d/87.pdf (last access: 8 April 2025), 2016a. a
Winkler, G., Wagner, T., Pauritsch, M., Birk, S., Kellerer-Pirklbauer, A., Benischke, R., Leis, A., Morawetz, R., Schreilechner, M. G., and Hergarten, S.: Identification and assessment of groundwater flow and storage components of the relict Schöneben Rock Glacier, Niedere Tauern Range, Eastern Alps (Austria), Hydrogeol. J., 24, 937–953, https://doi.org/10.1007/s10040-015-1348-9, 2016b. a
Winkler, M., Schellander, H., and Gruber, S.: Snow water equivalents exclusively from snow depths and their temporal changes: the Δsnow model, Hydrol. Earth Syst. Sci., 25, 1165–1187, https://doi.org/10.5194/hess-25-1165-2021, 2021a. a
Winkler, G., Wagner, T., and Krainer, K.: Wasserwirtschaftliche Aspekte von Blockgletschern in Kristallingebieten der Ostalpen – Speicherverhalten, Abflussdynamik und Hydrochemie mit Schwerpunkt Schwermetallbelastungen [Water resources management issues of rock glaciers in alpine catchments of the Eastern Alps – storage capacity, flow dynamics and hydrochemistry in particular heavy metal pollution], Beiträge zur Hydrogeologie, 63, 65–98, 2021b. a
Woo, M.-K.: Sustaining Groundwater Resources: A Critical Element in the Global Water Crisis, chap. Linking Runoff to Groundwater in Permafrost Terrain, Springer Netherlands, Dordrecht, 119–129, https://doi.org/10.1007/978-90-481-3426-7_8, 2011. a, b
Woo, M.-k.: Permafrost Hydrology, Springer Berlin Heidelberg, https://doi.org/10.1007/978-3-642-23462-0, ISBN 9783642234620, 2012. a
Woo, M.-k. and Xia, Z.: Effects of hydrology on the thermal conditions of the active layer: Paper presented at the 10th Northern Res. Basin Symposium (Svalbard, Norway – 28 Aug./3 Sept. 1994), Hydrol. Res., 27, 129–142, https://doi.org/10.2166/nh.1996.0024, 1996. a
Yoshikawa, K., Schorghofer, N., and Klasner, F.: Permafrost and seasonal frost thermal dynamics over fifty years on tropical Maunakea volcano, Hawai'i, Arct. Antarct. Alp. Res., 55, 2186485, https://doi.org/10.1080/15230430.2023.2186485, 2023. a
Zhu, M., Wang, J., Ivanov, V., Sheshukov, A., Zhou, W., Zhang, L., Mazepa, V., Sokolov, A., and Valdayskikh, V.: An analytical model of active layer depth under changing ground heat flux, J. Geophys. Res.-Atmos., 129, e2023JD039453, https://doi.org/10.1029/2023JD039453, 2024. a