the Creative Commons Attribution 4.0 License.
the Creative Commons Attribution 4.0 License.
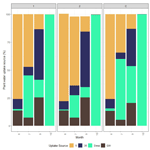
Seasonal shifts in depth-to-water uptake by young thinned and overstocked lodgepole pine (Pinus contorta) forests under drought conditions in the Okanagan Valley, British Columbia, Canada
Emory C. Ellis
Robert D. Guy
Xiaohua A. Wei
As drought and prolonged water stress become more prevalent in dry regions under climate change, preserving water resources becomes a focal point for maintaining forest health. Forest regeneration after forest loss or disturbance can lead to overstocked juvenile stands with high water demands and low water-use efficiency. Forest thinning is a common practice with the goal of improving tree health, carbon storage, and water use while decreasing stand demands in arid and semi-arid regions. However, little is known about the impacts of stand density on seasonal variation in depth-to-water uptake or the magnitude of the effect of growing season drought conditions on water availability. Existing reports are highly variable by climatic region, species, and thinning intensity. In this study, stable isotope ratios of deuterium (δ2H) and oxygen (δ18O) in water collected from various soil depths and from branches of lodgepole pine (Pinus contorta) under different degrees of thinning (control: 27 000 stems per hectare; moderately thinned: 4500 stems per hectare; heavily thinned: 1100 stems per hectare) over the growing season were analyzed using the MixSIAR Bayesian mixing model to calculate the relative contributions of different water sources in the Okanagan Valley in the interior of British Columbia, Canada. We found that under drought conditions the lodgepole pine trees shifted their depth-to-water uptake through the growing season (June to October) to rely more heavily on older precipitation events that percolated through the soil profile when shallow soil water became less accessible. Decreased forest density subsequent to forest thinning did not cause a significant difference in the isotopic composition of branch water but did cause changes in the timing and relative proportion of water utilized from different depths. Thinned lodgepole pine stands were able to maintain water uptake from 35 cm below the soil profile, whereas the overstocked stands relied on a larger proportion of deep soil water and groundwater towards the end of the growing season. Our results support other findings by indicating that, although lodgepole pines are drought-tolerant and have dimorphic root systems, they do not shift back from deep water sources to shallow soil water when soil water availability increases following precipitation events at the end of the growing season.
- Article
(2323 KB) - Full-text XML
-
Supplement
(373 KB) - BibTeX
- EndNote
As forests recover after harvesting, carbon and water demands change, and future climate projections of increased drought severity will further complicate biogeochemical cycling and carbon–water trade-offs (Giles-Hansen et al., 2021; Wang et al., 2019). Overpopulated regenerating stands can add further stress on ecosystems: for example, light competition in dense juvenile stands increases stand water demands by driving vertical growth and canopy cover (Liu et al., 2011). To mitigate this stress, management strategies such as systemic thinning of high-density juvenile stands have been shown to promote forest regeneration while decreasing competition and providing the remaining vegetation with increased light availability, rooting space, nutrient access, and space for horizontal branch growth (Giuggiola et al., 2016). Over a variety of forest ecosystems, reductions in stand density increase light availability, tree water use, carbon storage, and water-use efficiency (an indication of improved tree health) and decrease stand water use, reducing the intensity of water stress under drought conditions (Belmonte et al., 2022; Fernandes et al., 2016; Giuggiola et al., 2016; Liu et al., 2011; Manrique-Alba et al., 2020; Molina et al., 2012; Park et al., 2018; Sohn et al., 2012, 2016; Wang et al., 2019). Because the primary goal of forest thinning is to decrease stand water use and increase productivity, papers reporting the effects of this management strategy often focus on changes in carbon storage, tree growth, transpiration, and water-use efficiency (Giuggiola et al., 2016; Manrique-Alba et al., 2020; Park et al., 2018; Sohn et al., 2016). However, few studies have reported sources of water use for vegetation water uptake and shifts in depth-to-water uptake in association with thinning treatments in overstocked naturally regenerating forests, particularly under drought conditions.
Quantifying stand water use is imperative for predicting the future of water availability in our ecosystems. However, various studies indicate that trees do not always use the most recent precipitation and that vegetation can utilize different sources of water at different soil depths, depending on availability or stress (Dawson, 1996; Grossiord et al., 2017; Wang et al., 2017). Many studies also report the depth-to-water uptake of various species and the relationship between co-existing species and shared water sources (Andrews et al., 2012; Brinkmann et al., 2019; Grossiord et al., 2017; Langs et al., 2020; Liu et al., 2015; Maier et al., 2019; Meinzer et al., 2007; Sánchez-Pérez et al., 2008; Szymczak et al., 2020; Wang et al., 2017; Warren et al., 2005). In water-limited regions such as arid and semi-arid landscapes, some species have adapted to derive water from various depths over time depending on seasonal water variability, indicating higher ecological plasticity and drought tolerance (Langs et al., 2020; Wang et al., 2017). Understanding where in the soil profile plants obtain water, over prolonged dry periods and at different stand densities, is essential for assessing the impact of forest thinning and the relative importance of different seasonal water sources during shifts in water availability in arid regions and under future climate conditions (Evaristo et al., 2015; Prieto et al., 2012; Sohn et al., 2016). The implications of depth-to-water uptake and seasonal changes in water utilization, in conjunction with water-use efficiency, can emphasize the importance of the timing and volume of precipitation events and the primary contributors to vegetation water use.
Stable isotope ratios can be used as powerful natural tracers to identify distinct water sources such as rainfall, snow, and groundwater (Brinkmann et al., 2018; Lin and Sternberg, 1993; Sprenger et al., 2017; Stumpp et al., 2018). The isotopic signature of precipitation events is altered by elevation, temperature, and evaporative fractionation, creating distinctive layers within the soil profile (Kleine et al., 2020; Sprenger et al., 2017; Stumpp et al., 2018). More specifically, soil water reflects precipitation events as they infiltrate through the soil layer under the influence of evaporative fractionation until they mix with older soil water and groundwater and create individualized water isotopic signatures throughout the soil profile (Andrews et al., 2012; Brinkmann et al., 2018; Dawson, 1996; Sprenger et al., 2017; Stumpp et al., 2018). The isotopic composition of plant water can correspond to the water uptake depth in the soil profile (Brinkmann et al., 2019; Langs et al., 2020; Meinzer et al., 2007; Stumpp et al., 2018; Wang et al., 2017). Due to these unique characteristics, stable water isotopes have been used by researchers to assess sources of water used by plants and their possible shifts under altered environmental conditions (Evaristo et al., 2015; Flanagan and Ehleringer, 1991; Meinzer et al., 2001; Stumpp et al., 2018).
Lodgepole pine (Pinus contorta) is an early successional montane conifer with a deep tap root, fine roots in shallow soil layers, and an adventitious rooting system, which allow this species to access water throughout the soil profile (Fahey and Knight, 1986; Halter and Chanway, 1993). Depending on the species, root structures have two main components, i.e., lateral roots to exploit soil near the surface and, in species with dimorphic root systems, sinker roots or a well-developed tap root to reach deeper soil water or groundwater when surface water is limited. Species with dimorphic rooting systems can access water from different depths in the soil profile, depending on the soil moisture content and water availability, making them more resilient to water scarcity or prolonged drought conditions (Dawson, 1996; Meinzer et al., 2013). Wang et al. (2019) studied the short-term effects of thinning overstocked juvenile (16-year-old) lodgepole pine stands in the Upper Penticton Creek Watershed, British Columbia, Canada, and found a significant positive relationship between growth and water use from decreased stand density and that heavily thinned treatments showed the most drought resistance. Andrews et al. (2012) compared water uptake strategies between Douglas fir (Pseudotsuga menziesii (Mirb.) Franco) and lodgepole pine in southern Alberta and found that lodgepole pines are able to minimize seasonal variations in stem water potential and that tap roots are deep enough to access groundwater. These findings are consistent with other literature reporting that decreased stem density can improve water-use efficiency and that conifer trees can access water from different depths depending on moisture availability (Meinzer et al., 2007; Warren et al., 2005). The literature therefore indicates that lodgepole pines can access water from different soil layers, even under extreme or prolonged drought conditions, but little is known about the shifting of water use under different stand densities as a result of thinning treatments and drought conditions.
In this study, we build on the research of Wang et al. (2019), which looked at the effects of thinning on water-use efficiency during a drought year and a non-drought year by analyzing the stable isotope ratios (δ2H and δ18O) of soil and xylem water to evaluate at which depths overstocked and thinned stands access water over a growing season to further our understanding of the ecosystem-level impacts of thinning as a management strategy. We hypothesized that lodgepole pine primarily relies on spring snowmelt, but reductions in shallow source water during the growing season would drive trees to utilize deeper sources of water as the season progressed. We also hypothesized that decreased stand density (thinning) would increase shallow soil evaporation due to decreased canopy cover but also decrease competitive limitations in tree rooting zones so that at lower densities trees could better maintain mid-level soil water uptake. Through a detailed partitioning of tree water sources, we can better understand how lodgepole pine uses water, estimate the proportional dependence of lodgepole pine on specific source waters, and determine whether thinning affects tree water use and uptake strategies under drought conditions.
2.1 Study site
The study was conducted in the Upper Penticton Creek experimental watershed (UPC) northeast of Penticton in the interior of British Columbia, Canada (49°39′34′′ N, 119°24′,34′′ W). The site elevation is approximately 1675 m with steep, rocky terrain and a southern aspect (Wang et al., 2019). The Luvisol soils were formed from granite; the texture is coarse sandy loam and is well drained with a low water-holding capacity (Hope, 2011; Winkler et al., 2021; Winkler and Moore, 2006). The biogeoclimatic region is the Engelmann spruce–subalpine fir zone with cold, snowy conditions from November to early June and seasonal drought conditions during the summer months from June to October (Coupe et al., 1991; Wang et al., 2019). This research site was initially established as a paired watershed experiment in the early 1980s to quantify the impact of forest harvesting on water resources (Creed et al., 2014; Moore and Wondzell, 2005; Winkler et al., 2021).
The juvenile thinning experiment began in 2016, when 16-year-old, evenly aged, and regenerating lodgepole pine stands were thinned to different densities than a control (control C: 27 000 stems per hectare, T1: 4500 stems per hectare, and T2: 1100 stems per hectare), where C represents the control stands, T1 represents the moderately thinned stands, and T2 represents the heavily thinned stands (Fig. 1). The three treatments were repeated across the three replicate blocks. Each block was 75 m long and 25 m wide, with three 20 m2 plots and 5 m between the treatment plots. After the initial thinning, all debris was left on site.
2.2 Climate and soil moisture monitoring
Climate stations (HOBO weather station, Onset Computer, Bourne, MA, USA) were deployed across Block-1 treatments and have measured meteorological data since 2016 (ambient temperature, relative humidity (RH), wind speed, precipitation, and solar radiation) in 10 min intervals. From these data, we calculated the daily vapor pressure deficit (VPD) as well as daily and monthly potential evapotranspiration (PET) (Flint and Childs, 1991; Russell, 1960; Streck, 2003). Recorded historical precipitation (1997–2008) was acquired from a long-term climate station in a lodgepole pine forest in the 241 experimental watershed (climate station P7) (Moore et al., 2021).
Rainfall and temperature data from Block 1 were related to historical data to calculate the monthly dryness (), standardized precipitation index (SPI), and standardized precipitation evapotranspiration index (SPEI) (Table S1 in the Supplement) (Beguería et al., 2014; Stagge et al., 2014; Wu et al., 2005). In the middle of the growing season in 2021, four soil moisture probes (HOBO TEROS 11 soil moisture and temperature probes) were deployed in each treatment in Block 1 to measure changes in soil moisture and temperature at 5 and 35 cm at 15 min increments (n=12).
2.3 Sample collection
We sampled three trees per treatment across the three blocks and three trees in an adjacent mature plot south of the study site four times over the 2021 growing season in approximately 6-week intervals (11–12 June, 21–22 July, 10–11 September, and 7–8 October) around noon to capture the peak transpiration time (Table 1). We used a pole pruner to cut a mid-canopy branch in the live crown. We peeled the bark off branch segments with no needle coverage to remove outer bark and phloem, placed them in 10 mL glass tubes that then had Parafilm® wrap covered in aluminum foil, and set them in a cooler until the end of the day, when they were transferred to a freezer at −18 °C. During the last two sampling periods, some trees had red needles, likely an indication of dryness or higher temperatures from an early growing season heat dome that began in June.
Table 1Overview of the branch, soil, and precipitation samples collected over the four sampling periods during the 2021 growing season and additional campaigns to collect groundwater and stream water.
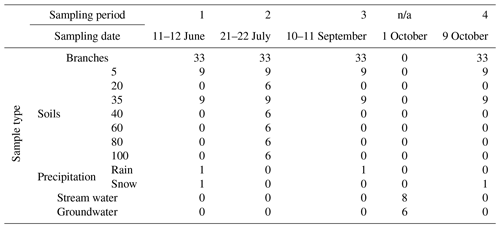
n/a stands for not applicable.
Soil samples were collected horizontally from 40 cm soil pits randomly dug within each treatment plot at depths of 5 and 35 cm from the surface from June to October 2021. Large rocks were removed from the profile. We conducted soil ribbon field tests to ensure that the clay composition was less than 10 % (soil ribbons were less than 20 mm in length). Soils were taken directly from the pit, sealed in freezer seal bags, and then frozen until cryogenic distillation for water extraction. In July, 1 m pits were dug. From the vertical pit, samples were collected in 20 cm increments to determine the depth of tree water access. After samples were collected, the larger rocks and soils were used to fill the pits. We assumed that the isotopic signature of soil water below 40 cm would be similar throughout the growing season and would be representative of deep soil water. Soil samples were stored in a freezer at −18 °C until cryogenic distillation.
Precipitation samples were collected cumulatively over individual field collection days where precipitation was present (Table 1). Snow from a late-spring event was collected on 11 June to represent snow water isotopic composition during the sublimation and melt periods of early 2021. Another snow event was collected on 11 October during active snowfall. A rain event was collected on 10 September. Groundwater and stream samples were collected from the creek 241 watershed in early October 2021 at the beginning of the seasonal hydraulic recovery period (Table 1). Groundwater was collected using a hand pump. Groundwater and stream water samples were collected at the end of the growing season as stream beds were dry and groundwater was inaccessible during the dry period. Once the well had been pumped and cleared, 10 mL glass test tubes were rinsed with groundwater three times before being filled. Precipitation, groundwater, and stream samples were collected in 10 mL glass test tubes, sealed with Parafilm® and foil, and stored in a fridge at 4 °C.
2.4 Cryogenic extraction and isotopic analysis
Before extraction, branch samples remained sealed and were weighed in the glass test tubes used for field collection. Branches remained in the test tubes until cryogenic distillation was complete to ensure that any liquid water lost from the branch to the test tube was contained in the extract. Soil samples were mixed in the Ziploc® bag, weighed, and transferred to a glass round-bottom flask. For stable isotope analysis, water was extracted from stem and soil samples using cryogenic distillation (Orlowski et al., 2013; Pearcy et al., 2012). The test tube and branch sample segment of the line were immersed in liquid nitrogen for 10 min until frozen (Chillakuru, 2009). The soil sample size for extraction was roughly determined based on the expected moisture of the frozen sample and soil moisture readings from continuous measurements in the field. Soils were frozen for 45 min in a 500 mL round-bottom flask using a dry-ice and 95 % ethanol mixture before pumping out the air. Frozen samples were pumped down to 60 mTorr, not disturbing the sample (Tsuruta et al., 2019). The vacuum-sealed extraction unit was detached from the pump and transferred to a bath of boiling water. The extraction tube was submerged in liquid nitrogen. Branch samples were set to distill for 1 h and soil samples for 2 h or until the tubing was clear to ensure that all mobile and bound source water was extracted (Orlowski et al., 2013; Tsuruta et al., 2019; Vargas et al., 2017; West et al., 2006). As reviewed by Allen and Kirchner (2022), the cryogenic vacuum distillation of water from plant tissues and soils can cause systematic biases in the measurements of δ2H. The degree of extraction bias varies depending on species and soil type (Allen and Kirchner, 2022). In contrast, the bias in δ18O values is close to zero (Allen and Kirchner, 2022). Reported biases in δ2H average about −6.1 ‰ for xylem water and −4 ‰ for water extracted from sandy soils, such as the soils sampled here, which are of a similar magnitude (Allen and Kirchner, 2022). Therefore, although we used cryogenic vacuum distillation to extract water from xylem and soil media, potential systematic bias introduced during the extraction process was treated as negligible, as all sources we identified had a difference in δ2H of greater than 4 ‰ (with the minimum distance being 14 ‰ between groundwater samples and deep soil water), minimizing any major effects on partitioning calculations.
The volume of the branch water extracted ranged from 1 to 3 mL, depending on the size of the branch sample. The total extracted water varied, depending on the mass of the initial sample. The volume of soil water extract ranged from 1 to 7 mL, depending on the size of the sample prepared for extraction. Samples were also weighed after extraction and compared to oven-dried samples to ensure that distillation was complete. Water extracted from branch and soil samples accounted for 47.9±3.2 % and 9±6 % of the mean sample weight ± standard deviation.
All samples were pipetted and sealed into glass vials with screw tops and shipped to the University of California Davis Stable Isotope Facility (Davis, CA, USA) for 18O and 2H analysis using headspace gas equilibration on a GasBench-II interfaced to a Delta Plus XL isotope-ratio mass spectrometer (Thermo-Finnigan, Bremen, Germany) normalized to a range of secondary reference waters calibrated against three IAEA standard waters. Precision was less than or equal to 2.0 ‰ for δ2H and 0.2 ‰ for δ18O. Results were returned in the delta notation, expressing the isotopic composition of each sample as a ratio in parts per thousand relative to VSMOW (Vienna Standard Mean Ocean Water), where
Sample extract was located in an isotope biplot and compared to the Global Meteoric Water Line (GMWL) along with the Okanagan Meteoric Water Line (OMWL) (δ2H = 6.6 (δ18O) − 22.7) and a local evaporative line (LEL) (δ2H = 5 (δ18O) − 48.4) calculated for the Okanagan Valley by Wassenaar et al. (2011). The LEL is a linear regression that indicates the departure of water sources from the OMWL to indicate the degree of evaporative processes fractionating the isotopic composition of water sources or variance in the isotopic composition of seasonal precipitation events.
One extreme outlier of B1C at the depth of 20 cm was removed before analysis: the high δ2H and δ18O values were likely due to contamination or incomplete cryogenic distillation. To test the variance between thinning treatments, block replicates, sampling periods, and soil depth, we first tested the assumption of normality in the subsets using the Shapiro–Wilk test and found that all subgroups were approximately normally distributed. Repeated-measure ANOVA was used to compare the effects of date and treatment on δ2H and δ18O in branches, soils, and groundwater to determine whether changes in lodgepole pine uptake patterns occurred over time, whether soil signatures varied between different depths (0–100 cm and groundwater) and densities, and whether thinning juvenile stands changed seasonal shifts. All statistical analysis was conducted in R Studio (version 1.3.1073) using the appropriate tests to determine site distinctions and seasonal variability in depth to uptake (R Studio Team, 2020).
2.5 MixSIAR model scenarios
Process-based models (PBMs) with a Bayesian approach integrate other processes or existing information as priors, allowing for a more informed approach than a simple linear model (Ogle et al., 2014). To accurately partition potential lodgepole pine water sources, we used the MixSIAR modeling package, a Bayesian mixing model (BMM) based on the Markov chain Monte Carlo (MCMC) method (Langs et al., 2020; Stock and Semmens, 2016; Stock et al., 2018 p. 201; Wang et al., 2017, 2019). The MixSIAR modeling package was selected over previous iterations of the dual-isotope BMM (SIAR and Simmr) and other partitioning models because of the accuracy of the analysis of covariates and the ability of the model to include source-specific uncertainties and discrimination factors (Stock et al., 2018; Wang et al., 2017). We partitioned potential water sources for five different scenarios using a combination of single- and dual-isotope approaches and different potential sources: scenario 1 – single-isotope δ18O and two sources at depths 5 and 35 cm; scenario 2 – single-isotope δ2H and two sources at depths 5 and 35 cm; scenario 3 – dual-isotope and two sources at depths 5 and 35 cm; scenario 4 – dual-isotope and three sources at depths 5, 35, and 45–100 cm; scenario 5 – dual-isotope and three sources – 5 cm, 35–100 cm, and groundwater; and scenario 6 – four dual-isotope sources – 5, 35, and 45–100 cm and groundwater. In scenarios using deep soil water (35–100 cm depths), the isotopic composition was calculated as a weighted average between seasonally collected soil water from depth 35 cm and average soil water at depths collected in 202 cm intervals during the early growing season (n=38 per season). There were no source concentration dependencies, and the discrimination was set to zero for both isotopes in the analysis. The run length of the MCMC method was set to “normal” (chain length = 100 000; burn = 50 000; thin = 50; chains = 3). The Gelman–Rubin and Geweke diagnostic tests included in the model package were used to determine convergence (Gelman–Rubin score < 1.01). Scenarios that did not converge were run again with a longer runtime (chain length: 300 000; burn: 200 000; thin: 100; chains = 3). No priors were used, so each water source was considered equally (α=1).
3.1 Climate and soil water content
The ambient temperature peaked in the moderately thinned plot (T1) on 29 June, with a maximum temperature of 36.3 °C in an abnormally hot and dry summer (Fig. 2). The relative humidity and VPD recorded in T1 showed the most variability and the highest evaporative capacity during July. Atmospheric water vapor was higher in late September and October, when precipitation was more frequent and the watershed began to exhibit traits of hydrological recovery (Fig. 2). One indication of increased water availability was increased soil moisture at the depths of 5 and 35 cm and more groundwater recharge in October (Fig. 3). There was 17.5 mm of precipitation from 16 to 18 September that infiltrated to at least 35 cm below the soil surface, along with subsequent rainfall events that likely infiltrated past the 35 cm sample depth, changing the isotopic composition of deep soil water from what was measured during the deep pit sampling in July).
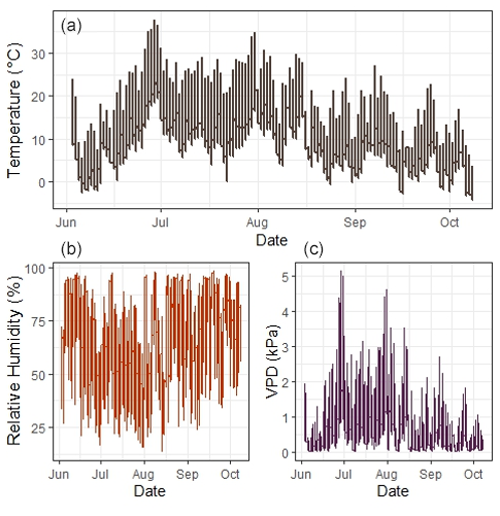
Figure 2The 15 min measurements of (a) atmospheric temperature (°C), (b) relative humidity (%), and (c) vapor pressure deficit (VDP) (kPa).
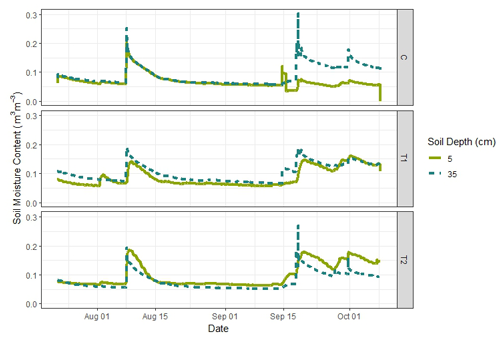
Figure 3Average in situ continuous measurements (15 min interval) of soil water content (m3 m−3) from the control, moderately thinned, and heavily thinned stands in Block 1.
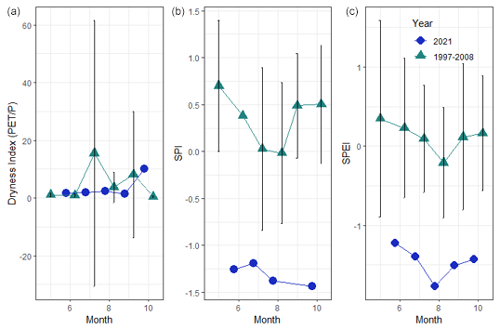
Figure 5From left to right: the dryness index (monthly PET using the Thornthwaite method divided by mean monthly precipitation) from June to October 2021 and historic climate data from 1997 to 2008, including the standard error for the historic climate data; the standard precipitation index (SPI) was calculated given 3-month intervals for the study period between June and October 2021, together with historic (1997–2008) climate data and the standard error for the historic climate data; and the standardized precipitation evapotranspiration index (SPEI) for 3-month intervals for the study period between June and October 2021 and historic (1997–2008) climate data, including the standard error for the historic climate data.
3.2 Water-stable isotopes
The biplot of sample isotopic composition shows the distribution and effect of isotopic fractionation on source water isotope ratios of samples collected during the 2021 field season. Field-collected samples were compared to the OMWL (Wassenaar et al., 2011). The slopes for branch and soil water were less steep than those of the OMWL and the intercepts were more negative, indicating that evaporative fractionation contributed to the isotopic composition of these pools at the UPC (Fig. 6). The soil samples seemed to follow the LEL produced by Wassenaar et al. (2011) for the region, indicating similar evaporative fractionation effects. Branch water more closely followed the OMWL than soils, suggesting that most samples consisted of water that was accessed from deeper in the soil profile and that had infiltrated past the evaporative front. Precipitation samples collected during the field season fell along the OMWL (Wassenaar et al., 2011). The δ2H and δ18O of the 11 June rainfall event were −127.5 ‰ and −13.03 ‰, respectively. The September rainfall event was much more enriched, with a δ2H of −38.4 ‰ and a δ18O of −2.89 ‰ (Fig. 6). The snowfall collected on 7 October more closely resembled the lighter and colder June precipitation event.
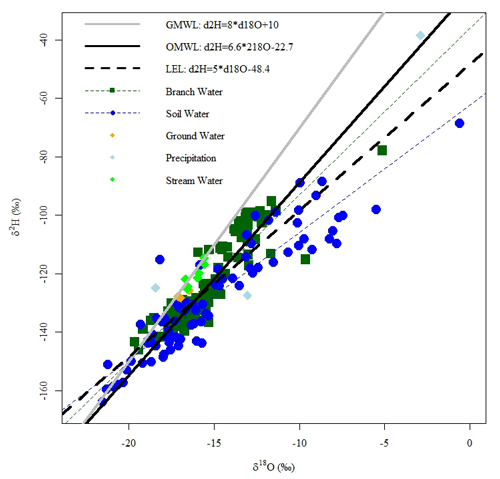
Figure 6Biplot of δ18O and δ2H, including all branch, soil, stream water, groundwater, and precipitation samples collected over the 2021 study period outlined in Table 1 along with the Global Meteoric Water Line (GMWL), the Okanagan Meteoric Water Line (OMWL), and the local evaporative line (LEL) developed by Wassnaar et al. (2011). Linear regressions are also plotted for branch water and soil water to indicate deviations in the slope and intercept from the OMWL and LEL. The relationship between δ18O and δ2H in soil and branch water shows comparative ranges but more variation among the soil water samples, likely due to changes in precipitation signatures.
3.2.1 Soil moisture and seasonal water composition
Soil moisture probes and percent soil water content from samples collected for isotopic analysis were compared between treatments and deployment depths. The water content of the soil samples was highest in June (21.5 % at 5 cm and 21.6 % at 35 cm) because of high snowmelt and early spring precipitation, while soils were driest in September (6.32 % at 5 cm and 6.19 % at 35 cm). Continuous soil moisture measurements showed that soil water began to increase in mid-September as precipitation became more frequent, daily solar radiation decreased, and water percolated into deeper soil layers. There were significant differences in the continuously measured soil moisture by depths, treatments, and months, respectively (5–35 cm) (depth: F=3545.9, ; treatment: F=1883.3, ; month: F=3359.8, ) (Fig. 7), but the soil water content of samples for isotopic analysis only varied significantly by month (August–October) (F=22, ).
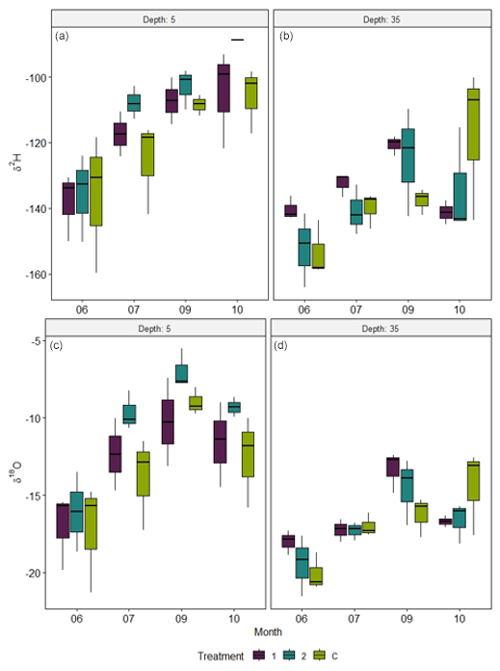
Figure 7Boxplots of the soil water δ2H (a, b) and δ18O (c, d) at 5 cm (a, c) and 35 cm (b, d) depths collected four times over the growing season from each treatment and block. The mean, interquartile ranges, and standard deviation are indicated for each treatment in each month. There was a significant difference in the isotopic composition of water between months by treatment and depth, indicating changes in the water isotopic signature due to evaporation or precipitation.
Soil isotopic results were broken into two datasets to analyze the variation in isotopic composition over time and between treatments, and then a profile of isotopic variance with depth was constructed. Soil water δ2H and δ18O varied significantly by depth (δ2H: ; δ18O: ), being higher in the shallow soils than deeper in the profile (Fig. 7a and c). δ2H varied significantly across months (), but not between July and September and between September and October. δ18O also varied significantly across months (), except when directly comparing July to October and September to October. Despite treatment differences in soil moisture (Fig. 4), there were no statistically significant treatment differences in the isotopic composition of soil water at either depth. In June, the mean soil water δ18O at 5 cm was ‰, while the δ2H was ‰; at 35 cm, the δ18O was ‰ and the δ2H was ‰. Both δ18O and δ2H increased more during the growing season at 5 cm than at 35 cm, and with more variability (Fig. 7). In September, δ18O and δ2H at 5 cm were −8.75 ‰ and −106.23 ‰, and at 35 cm they were −14.71 ‰ and −127.64 ‰, respectively, suggesting that soil isotopic composition nearer the soil surface follows trends in precipitation samples, being most enriched by O18. By October, δ18O and δ2H at 5 cm reflected more recent precipitation events, indicating that water availability in shallow soils began to increase.
From the isotopic soil profile, there were three significant groupings of isotopic composition (p<0.05): shallow soil water (5–20 cm), deep soil water (35–100 cm), and groundwater. Mean groundwater collected at the end of the growing season most closely resembled spring and fall snowfall events. The mean δ18O of groundwater was ‰, which resembles that in the soil profile, but the mean δ2H was slightly higher than that of soil water (n=4). This isotope fractionation may be due to interactions with bound soil water and soils as the water infiltrates through the vadose zone, but the spread of values as potential uptake sources was greater than any predicted bias from cryogenic vacuum extraction. Therefore, groundwater was included in the model as a isotopically distinct potential source of lodgepole pine water use (Allen and Kirchner, 2022; Vargas et al., 2017).
The more negative values for both δ18O and δ2H with soil depth indicate that snowmelt is the main source of water to the deep unsaturated zone and that enriched summer precipitation does not infiltrate deeper soil layers (Fig. 8).
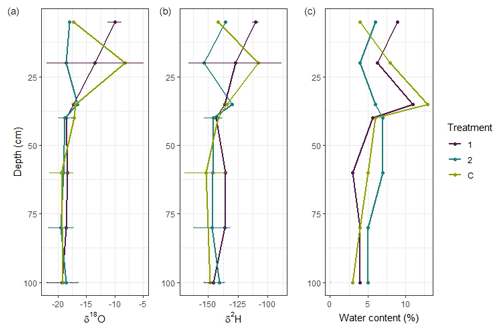
Figure 8Vertical isotopic profiles and gravimetric soil water content from treatments in Block 2 and samples collected in mid-July, where panel (a) shows the vertical changes in δ18O for each treatment, panel (b) shows the vertical changes in δ2H for each treatment, and panel (c) shows the change in the gravimetric water content as a percentage of the total soil weight.
3.2.2 Isotopic variability in branch xylem water
Branch xylem for each treatment across the three blocks and the adjacent mature stand was compared for each sampling period. All the treatments closely resembled the mature stand in both δ18O and δ2H. There were no statistically significant differences in both δ18O and δ2H of xylem water across thinning treatments; there was, however, significant variation over time (δ18O: ; δ2H: ). More specifically, δ18O and δ2H of xylem water varied by month for all the months collected, except for between June and September and between July and September (Fig. 9). Because the isotopic composition of xylem water showed significant change over the growing season but did not follow the same seasonal trends as soil water, the trees were likely changing their primary water source within the soil profile.
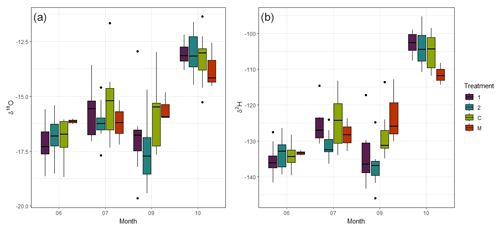
Figure 9A boxplot showing the branch mean, interquartile range, and standard deviation for (a) δ18O and (b) δ2H by month and treatment for the control (C), lightly thinned (T1), heavily thinned (T2), and mature (M) stands. Branch water was highest in October despite the treatment effects. Mature trees were used as a reference for the isotopic composition of lodgepole pines over time but were not considered in the model of changes in the depth-to-water uptake over time. There was no statistically significant difference in δ18O and δ2H between the treatments, but each treatment varied significantly by month, with the highest concentration of heavy isotopes in October.
3.3 Partitioning xylem source water and seasonal fluxes using MixSIAR
Of the six scenarios considered, scenarios 1, 2, and 6 approached the Gelman–Rubin diagnostic (less than 1.05) with a runtime set to normal (chain length: 100 000; burn: 50 000; thin: 50; chains: 3), which indicates that they were the closest of all the scenarios to reach convergence (Table S2 in the Supplement). Out of the six potential scenarios, scenarios 4 (dual-isotope and 5, 35, and 45–100 cm soil water as sources) and 6 (dual-isotope and 5, 35, and 45–100 cm groundwater and soil water as sources) were rerun with the runtime set to “long” (chain length: 300 000; burn: 200 000; thin: 100; chains: 3) as they were hypothesized to provide the most representative results of water uptake partitioning from various depths. The Gelman–Rubin diagnostic for scenario 4 was 120, and for scenario 6 it was 17 when run for the long runtime, meaning that scenario 6 was closer to convergence but still greater than the convergence threshold.
The results of scenario 6 indicate that, in June, the trees in each treatment acquired the most water from the depth of 5 cm (C: 76 %; T1: 77 %; T2: 79 %) (Fig. 10). In July, shallow soil water was still the primary source of T1 and T2 at 47 % and 61 %, but C had 55 % of its water from 45 to 100 cm deep and only 33 % from 5 cm below the surface. By September, all the treatments acquired less than 15 % of their tree water from shallow soil. Lodgepole pine water use in treatments 1 and 2 was composed of approximately 48 % and 54 % from around 35 cm, while 72 % of the water in control stand trees was from 35 to 100 cm. By October, although the SPEI results indicate more moisture and less evaporative demand, scenario 6 indicated that all three treatments had the most water uptake from below 45 cm in the soil profile (Fig. 10). The results of the MixSIAR model support findings of branch water-stable isotope trends over the growing season, where the branch water started with mean δ18O and δ2H values of ‰ and ‰ in June, becoming slightly more enriched in July. There was a shift to a source with a higher concentration of lighter isotopes in September. Branch water was most enriched, with heavy isotopes in October, like shallow soil water, with mean δ18O and δ2H values of ‰ and ‰, respectively. However, the MixSIAR model does not account for potential changes in the isotopic composition of water from precipitation events from mid-September to mid-October. Additionally, we did not the consider extraction bias of the soil water sources or branch water in the MixSIAR model because the previously mentioned range between distinct sources is larger than the potential change in the isotopic signature during cryogenic distillation. The branch water in October was more enriched in heavy oxygen isotopes for each treatment than soil water at a depth of 35 cm and was more isotopically similar to soil water at 5 cm. Deuterium also followed a similar trend.
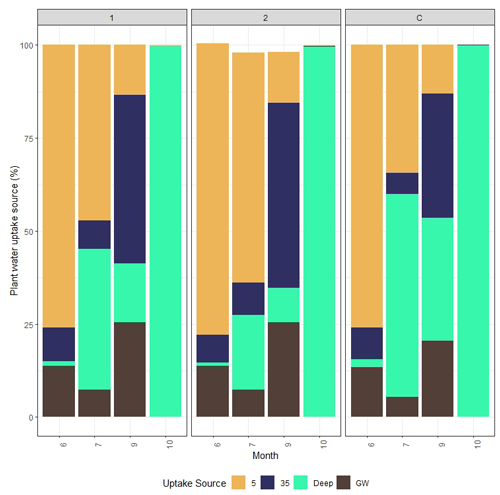
Figure 10Stacked bar charts showing the partitioned relative contribution of different sources of water in the soil profile by the MixSIAR model of scenario 6 with a long (chain length: 300 000; burn: 200 000; thin: 100; chains: 3) runtime. Scenario 6 considers both δ18O and δ2H to be tracers and 5, 35, and 45–100 cm and groundwater to be potential sources. Soil water isotopic composition at 5 and 35 cm changes monthly, whereas the concentrations of δ18O and δ2H are held constant for the 45–100 cm and groundwater sources. The results of this model indicate that there are significant changes in the depth-to-water uptake of lodgepole pines between June and October 2021 and that thinned trees can maintain a larger percentage of water uptake from shallow soil water longer than trees in the control stand.
4.1 Seasonal variability in soil water
Deep soil water showed a mixed gradient of older and more depleted water molecules deeper in the profile, indicating that deep soil water mainly originates from spring snowmelt during the summer months. Low-intensity and less-frequent summer precipitation events are evaporated out of the shallow soil layers and do not infiltrate past the evaporative front to recharge the unsaturated zone or groundwater. Although there was no statistically significant difference in the depth-to-water uptake by thinning treatments, the results of our isotopic analysis indicate that there was increased evaporative enrichment or a higher concentration of oxygen-18 in the shallow soils of the heavily thinned stand compared to the oxygen-18 concentrations in the moderately thinned and control stands (Fig. 7c). The muted enrichment in 18O around a depth of 35 cm in the soil indicates a mixing of the leftover summer precipitation with older and lighter water. Our results do not indicate that differences in soil exposure canopy coverage were effective enough to significantly affect the isotopic composition of soil water below 5 cm in depth.
4.2 Seasonal lodgepole pine water use
The literature utilizing stable water isotopic analysis to determine plant preferential water uptake in arid regions indicates that vegetation can utilize precipitation despite the temporal origin (Andrews et al., 2012; Brinkmann et al., 2019; Ehleringer et al., 1991). Seasonal water availability depends on precipitation, soil water-holding capacity and drainage, and evaporative loss (Gibson and Edwards, 2002; Kleine et al., 2020; Stumpp et al., 2018). Based on the seasonal shift in the isotopic composition, soil water at a depth of 5 cm was more enriched, with heavier isotopes over the growing season than at 35 cm due to more evaporative isotopic fractionation near the soil surface and a lack of rainfall intense enough to drive precipitation deeper into the soil profile before 16 September 2021 (Fig. 3). The effect of evaporative enrichment of the near-surface soil water was most obvious in July and September in the heavily thinned stand (T2). However, variability in branch isotopic composition did not follow the same trends. Our results indicate that lodgepole pine accesses water from multiple depths in the soil profile. Regardless of depth and forest density, spring snowmelt is the main source of lodgepole pine, as it infiltrates the vadose zone.
The MixSIAR isotopic partitioning model results from each of the six scenarios indicated a seasonal shift in the depth-to-water uptake of lodgepole pine, regardless of changes in stem density, over the growing season. At the beginning of the growing season, when snowmelt is more available at shallow depths and beginning to infiltrate through the soils, lodgepole pine obtains most of its water from snowmelt in shallow soils, with small contributions from other potential sources (<25 % of June water uptake in all treatments). Then, in July, the trees in the control treatment used less shallow soil water (34.3 % of plant water uptake from 5 cm below the soil profile), whereas the moderately thinned and heavily thinned plots maintained a greater proportion of shallow water uptake (47.1 % and 61.5 %, respectively). The mean δ18O and δ2H of branch water from each treatment in September had a higher concentration of lighter stable water isotopes than in July, and a larger proportion of tree water was 35–100 cm deep in the soil profile as shallow soils were dry from a lack of rainfall and surface soil evaporation. By September, the control stand was more dependent on deeper soil water and groundwater, with only 33.4 % of plant water uptake originating from 35 cm in the soil profile, whereas both thinning treatments maintained more than 45 % of the water uptake from 35 cm in the soil profile. In October, all the treatments were completely dependent on deep soil water, but it is likely that the isotopic profile of deep soil water sampled in July skewed the results. It is plausible that the trees began to rely on shallow soil water towards the end of the growing season, when soil water content increased. Further research is needed with more intensive sampling of deep soil water during the hydrological recharge period at the end of the growing season and beginning of senescence.
Local monitoring close to the study site indicated that the depth to groundwater stayed at least 6.5 m below the surface from August through the end of the study period. The continued use of deep soil water even during rewetting in late September and October suggests that the drought conditions suppressed topsoil water uptake but that deeper soil was sufficiently saturated to sustain root water uptake and tree function to limit groundwater uptake to less than 30 % for all treatments until the beginning of fall precipitation events recharging the saturated zone.
Our results indicate that lodgepole pine, like other pine species in arid regions, is flexible in its ability to access deep soil water and can change its depth-to-water uptake over time, depending on water availability (Brinkmann et al., 2018; Grossiord et al., 2017; Kerhoulas et al., 2013; Kleine et al., 2020; Moreno-Gutiérrez et al., 2011; Simonin et al., 2006; Sohn et al., 2014; Wang et al., 2021). Our results from seasonal changes in depth-to-water uptake by lodgepole pine support the findings of Andrews et al. (2012) on changes in lodgepole pine depth-to-water uptake in Alberta. Tree species native to arid regions exhibit a variety of adaptations to long-term drought stress and decreased water availability in the soil profile, e.g., deep tap roots, access to the water table, utilizing bound and mobile soil water, fine-root mortality, and hydraulic redistribution in ecosystems with low water-holding capacity (Amin et al., 2020; Brinkmann et al., 2018; Grossiord et al., 2017; Kerhoulas et al., 2013; Kleine et al., 2020; Langs et al., 2020; Meinzer et al., 2007; Prieto et al., 2012; Sohn et al., 2016; Wang et al., 2017, p. 201).
The literature is inconsistent across different biogeoclimatic regions and species with regards to the effects of thinning on stand dynamics that influence inter-tree competition for water resources or changes in depth-to-water uptake (Kerhoulas et al., 2013; Moreno-Gutiérrez et al., 2011; Sohn et al., 2016; Wang et al., 2021). We found no significant impact of forest thinning on depth-to-water uptake. However, our observation of seasonal shifts in depth-to-water uptake support the results of a study on the impacts of thinning intensity on 60-year-old Pinus halepensis Mill. in a semi-arid region of Spain, which concluded that forest thinning reduced competition for water resources but did not alter water uptake patterns (Moreno-Gutiérrez et al., 2011). Another study on the impact of thinning Pinus ponderosa Dougl. on depth-to-water uptake concluded that water was consistently more isotopically enriched in low-density stands, potentially due to prolonged evaporative fractionation in the soil profile, or that understory vegetation utilized more shallow water sources (Kerhoulas et al., 2013). The impact of forest thinning on stand and understory water use is highly variable and dependent on understory growth, canopy structure, water availability (when forest thinning is implemented), and the time since stem removal (Kerhoulas et al., 2013; Moreno-Gutiérrez et al., 2011; Sohn et al., 2016). More research is needed to discern whether lodgepole pine relies more on mobile or bound soil water, the extent of lodgepole pine rooting zones, which biogeochemical factors cause seasonal shifts in water uptake, and whether severe seasonal drought has a lasting effect on water uptake strategies during hydrological recovery (Simonin et al., 2007; Vargas et al., 2017).
4.3 Impacts of the drought and implications for future climate conditions
The 2021 growing season was an abnormally hot and dry period for the interior of British Columbia, with severe to exceptional drought conditions. Wang et al. (2019) found that thinning improved water-use efficiency, drought tolerance, and drought recovery by decreasing stand density and improving carbon storage. Our results support the finding that lodgepole pine trees can adjust to prolonged water scarcity, and overpopulated stands may be more resilient than the literature initially indicated. In fact, drought conditions over the study period likely intensified the change in xylem water isotopic composition over the growing season. However, the scope of this study did not include pre-drought seasonal water-use patterns or the impact of forest density on depth-to-water uptake during drought recovery. Because lodgepole pine depth-to-water uptake changes during prolonged dry growing season conditions, the trees are more reliant on winter snowpack and spring infiltration to recharge deeper source water below the evaporative front. One experiment on juniper (Juniperus monosperma (Engelm.) Sarg.) and pinion pine (Pinus edulis Engelm.) investigated the simultaneous stress of increased heat and decreased precipitation on depth-to-water uptake and found that extreme temperatures and decreased precipitation lead to less reversible embolism and more root death in surface soil levels, preventing trees from accessing shallow water sources if precipitation becomes more available late in the growing season (Grossiord et al., 2017). It is becoming more imperative to understand the climatic drivers of lodgepole pine water use and access as mean annual temperatures continue to rise, the seasonal frequency and intensity of precipitation change, and drought conditions become more severe. This study indicates that severe seasonal dryness pushes lodgepole pines to rely more on snowmelt while losing function in shallow roots. Our results are inconclusive in determining the depth-to-water uptake in September and October because of limited deep soil water measurements. However, increased annual temperatures and more variable precipitation patterns as a part of climate change projections are predicted to drive decreases in winter snowpack and could drive lodgepole pine stands, regardless of stem density, to rely on groundwater, influencing water availability and depth to groundwater. These projections could lead to prolonged interannual water scarcity along with seasonal water scarcity during the late growing season.
Lodgepole pine, across all treatments, was able to shift access from shallow soil water at the beginning of the growing season to deeper soil water as drought conditions intensified. The quick-draining and sun-exposed soils of the UPC do not retain small summer precipitation events, and these patterns are intensified in the shallow soil layer of the heavily thinned stand because decreased canopy cover can be directly related to increased soil evaporation. As a result, due to changes in water availability, lodgepole pine shifts to a more readily available source in the soil profile (Aranda et al., 2012; Prieto et al., 2012). Our findings support the literature, where lodgepole pine is a drought-tolerant species with dimorphic rooting systems allowing it to access water from varying depths in the soil depending on water availability (Andrews et al., 2012; Liu et al., 2011). Despite the ecological plasticity under extreme heat and low summer precipitation conditions, there was no statistically significant variance in depth-to-water use between overpopulated plots and thinned ones. Both thinned and unthinned lodgepole pine stands were able to access shallow soil water during the early months (June and July) and then switched to deeper soil water and a larger proportion of groundwater during September. Although there was no statistically significant difference in the isotopic composition of branch water for the different treatments, our results indicate that decreased stem density may lead to the prolonged use of soil water 35 cm below the surface during prolonged dry periods, which would decrease the dependency of lodgepole pine on shallow soil water and summer precipitation events and rather increase the dependency on deep soil water or groundwater fed by winter snow accumulation and spring snowmelt.
Future climate projections indicate hotter growing seasons and less precipitation (Allen et al., 2010). Further investigation is needed to discern how lodgepole pines, under different stand densities, use water during prolonged drought and drought recovery periods (Grossiord et al., 2017; Navarro-Cerrillo et al., 2019; Simonin et al., 2007; Sohn et al., 2016). From our findings, stand density did not prevent lodgepole pines from accessing soil water from various depths, but decreased stem density did result in lodgepole pines using soil water higher in the soil profile for longer under extremely dry conditions. Lodgepole pines indicate a strong level of drought tolerance and ability to access water under extreme heat conditions. If summer precipitation decreases, lodgepole pine in the interior of British Columbia can access deeper soil water from spring snowmelt. However, if snowpack and spring snowmelt begin to decrease, lodgepole pine may need to acclimate to these hydrological shifts.
The codes of the data analysis and plotting are available at https://doi.org/10.5281/zenodo.13975471 (Ellis, 2024) and are available upon request (ece58@nau.edu).
The supplement related to this article is available online at: https://doi.org/10.5194/hess-28-4667-2024-supplement.
ECE conceived the idea as part of their Master's research with XAW and performed the extractions with RDG. Analysis was primarily conducted by ECE with guidance from XAW and RDG. All the authors contributed to the manuscript.
The contact author has declared that none of the authors has any competing interests.
Publisher's note: Copernicus Publications remains neutral with regard to jurisdictional claims made in the text, published maps, institutional affiliations, or any other geographical representation in this paper. While Copernicus Publications makes every effort to include appropriate place names, the final responsibility lies with the authors.
This study was funded by the Ministry of Forests, Lands, Natural Resource Operations and Rural Development. The fieldwork was done with the assistance of Fiona Moodie. Cryogenic distillation was conducted at the University of British Columbia. Samples were sent to the Stable Isotope Facility at the University of California, Davis.
This research was supported by the Ministry of Forests, Lands, Natural Resource Operations and Rural Development (grant no. RE21NOR-029).
This paper was edited by Nadia Ursino and reviewed by Jose Ignacio Querejeta and two anonymous referees.
Allen, C. D., Macalady, A. K., Chenchouni, H., Bachelet, D., McDowell, N., Vennetier, M., Kitzberger, T., Rigling, A., Breshears, D. D., Hogg, E. H. (Ted), Gonzalez, P., Fensham, R., Zhang, Z., Castro, J., Demidova, N., Lim, J.-H., Allard, G., Running, S. W., Semerci, A., and Cobb, N.: A global overview of drought and heat-induced tree mortality reveals emerging climate change risks for forests, Forest Ecol. Manage., 259, 660–684, https://doi.org/10.1016/j.foreco.2009.09.001, 2010.
Allen, S. T. and Kirchner, J. W.: Potential effects of cryogenic extraction biases on plant water source partitioning inferred from xylem-water isotope ratios, Hydrol. Process., 36, e14483, https://doi.org/10.1002/hyp.14483, 2022.
Amin, A., Zuecco, G., Geris, J., Schwendenmann, L., McDonnell, J. J., Borga, M., and Penna, D.: Depth distribution of soil water sourced by plants at the global scale: A new direct inference approach, Ecohydrology, 13, e2177, https://doi.org/10.1002/eco.2177, 2020.
Andrews, S. F., Flanagan, L. B., Sharp, E. J., and Cai, T.: Variation in water potential, hydraulic characteristics and water source use in montane Douglas-fir and lodgepole pine trees in southwestern Alberta and consequences for seasonal changes in photosynthetic capacity, Tree Physiol., 32, 146–160, https://doi.org/10.1093/treephys/tpr136, 2012.
Aranda, I., Forner, A., Cuesta, B., and Valladares, F.: Species-specific water use by forest tree species: From the tree to the stand, Agr. Water Manage., 114, 67–77, https://doi.org/10.1016/j.agwat.2012.06.024, 2012.
Beguería, S., Vicente-Serrano, S. M., Reig, F., and Latorre, B.: Standardized precipitation evapotranspiration index (SPEI) revisited: parameter fitting, evapotranspiration models, tools, datasets and drought monitoring, Int. J. Climatol., 34, 3001–3023, https://doi.org/10.1002/joc.3887, 2014.
Belmonte, A., Ts. Sankey, T., Biederman, J., Bradford, J. B., and Kolb, T.: Soil moisture response to seasonal drought conditions and post-thinning forest structure, Ecohydrology, 15, e2406, https://doi.org/10.1002/eco.2406, 2022.
Brinkmann, N., Seeger, S., Weiler, M., Buchmann, N., Eugster, W., and Kahmen, A.: Employing stable isotopes to determine the residence times of soil water and the temporal origin of water taken up by Fagus sylvatica and Picea abies in a temperate forest, New Phytol., 219, 1300–1313, 2018.
Brinkmann, N., Eugster, W., Buchmann, N., and Kahmen, A.: Species-specific differences in water uptake depth of mature temperate trees vary with water availability in the soil, Plant Biol., 21, 71–81, https://doi.org/10.1111/plb.12907, 2019.
Chillakuru, D. R.: Towards locating and quantifying rrepiration in the soil and in the plant using a novel 18-oxygen labelling technique, MSc Thesis, University of British Columbia, https://doi.org/10.14288/1.0058469, 2009.
Coupe, R., Steward, A. C., and Wikeem, B. M.: Engelmann Spruce – Subalpine Fir Zone, in: Special report series 6: Ecosystems of British Columbia, BC Ministry of Forests, 223–236, 1991.
Creed, I. F., Spargo, A. T., Jones, J. A., Buttle, J. M., Adams, M. B., Beall, F. D., Booth, E. G., Campbell, J. L., Clow, D., Elder, K., Green, M. B., Grimm, N. B., Miniat, C., Ramlal, P., Saha, A., Sebestyen, S., Spittlehouse, D., Sterling, S., Williams, M. W., Winkler, R., and Yao, H.: Changing forest water yields in response to climate warming: results from long-term experimental watershed sites across North America, Global Change Biol., 20, 3191–3208, https://doi.org/10.1111/gcb.12615, 2014.
Dawson, T. E.: Determining water use by trees and forests from isotopic, energy balance and transpiration analyses: the roles of tree size and hydraulic lift, Tree Physiol., 16, 263–272, https://doi.org/10.1093/treephys/16.1-2.263, 1996.
Ehleringer, J. R., Phillips, S. L., Schuster, W. S. F., and Sandquist, D. R.: Differential utilization of summer rains by desert plants, Oecologia, 88, 430–434, https://doi.org/10.1007/BF00317589, 1991.
Ellis, E. C.: emory-ce/LodgepolePineWaterUseStrategies2021: Seasonal shifts in the depth-to-water uptake by young thinned and overstocked lodgepole pine (Pinus contorta) forests under drought conditions in the Okanagan Valley, British Columbia, Canada (Version v1), Zenodo [code and data set], https://doi.org/10.5281/zenodo.13975471, 2024.
Evaristo, J., Jasechko, S., and McDonnell, J. J.: Global separation of plant transpiration from groundwater and streamflow, Nature, 525, 91–94, https://doi.org/10.1038/nature14983, 2015.
Fahey, T. J. and Knight, D. H.: Lodgepole Pine Ecosystems, BioScience, 36, 610–617, https://doi.org/10.2307/1310196, 1986.
Fernandes, T. J. G., Del Campo, A. D., Herrera, R., and Molina, A. J.: Simultaneous assessment, through sap flow and stable isotopes, of water use efficiency (WUE) in thinned pines shows improvement in growth, tree-climate sensitivity and WUE, but not in WUEi, Forest Ecol. Manage., 361, 298–308, https://doi.org/10.1016/j.foreco.2015.11.029, 2016.
Flanagan, L. B. and Ehleringer, J. R.: Stable Isotope Composition of Stem and Leaf Water: Applications to the Study of Plant Water Use, Funct. Ecol., 5, 270–277, https://doi.org/10.2307/2389264, 1991.
Flint, A. L. and Childs, S. W.: Use of the Priestley-Taylor evaporation equation for soil water limited conditions in a small forest clearcut, Agr. Forest Meteorol., 56, 247–260, https://doi.org/10.1016/0168-1923(91)90094-7, 1991.
Gibson, J. J. and Edwards, T. W. D.: Regional water balance trends and evaporation-transpiration partitioning from a stable isotope survey of lakes in northern Canada, Global Biogeochem. Cy., 16, 10-1–10-14, https://doi.org/10.1029/2001GB001839, 2002.
Giles-Hansen, K., Wei, X., and Hou, Y.: Dramatic increase in water use efficiency with cumulative forest disturbance at the large forested watershed scale, Carb. Balance Manage., 16, 6, https://doi.org/10.1186/s13021-021-00169-4, 2021.
Giuggiola, A., Ogée, J., Rigling, A., Gessler, A., Bugmann, H., and Treydte, K.: Improvement of water and light availability after thinning at a xeric site: which matters more? A dual isotope approach, New Phytol., 210, 108–121, https://doi.org/10.1111/nph.13748, 2016.
Grossiord, C., Sevanto, S., Dawson, T. E., Adams, H. D., Collins, A. D., Dickman, L. T., Newman, B. D., Stockton, E. A., and McDowell, N. G.: Warming combined with more extreme precipitation regimes modifies the water sources used by trees, New Phytol., 213, 584–596, 2017.
Halter, M. R. and Chanway, C. P.: Growth and root morphology of planted and naturally-regenerated Douglas fir and Lodgepole pine, Ann. Forest Sci., 50, 71–77, https://doi.org/10.1051/forest:19930105, 1993.
Hope, G. D.: Clearcut harvesting effects on soil and creek inorganic nitrogen in high elevation forests of southern interior British Columbia, Can. J. Soil Sci., 89, 35–44, https://doi.org/10.4141/CJSS06032, 2011.
Kerhoulas, L. P., Koch, G. W., and Kolb, T. E.: Tree size, stand density, and the source of water used across seasons by ponderosa pine in northern Arizona, Forest Ecol. Manage., 289, 425–433, https://doi.org/10.1016/j.foreco.2012.10.036, 2013.
Kleine, L., Tetzlaff, D., Smith, A., Wang, H., and Soulsby, C.: Using water stable isotopes to understand evaporation, moisture stress, and re-wetting in catchment forest and grassland soils of the summer drought of 2018, Hydrol. Earth Syst. Sci., 24, 3737–3752, https://doi.org/10.5194/hess-24-3737-2020, 2020.
Langs, L. E., Petrone, R. M., and Pomeroy, J. W.: A δ18O and δ2H stable water isotope analysis of subalpine forest water sources under seasonal and hydrological stress in the Canadian Rocky Mountains, Hydrol. Process., 34, 5642–5658, https://doi.org/10.1002/hyp.13986, 2020.
Lin, G. and Sternberg, da S. L. L.: 31 – Hydrogen Isotopic Fractionation by Plant Roots during Water Uptake in Coastal Wetland Plants, in: Stable Isotopes and Plant Carbon-water Relations, edited by: Ehleringer, J. R., Hall, A. E., and Farquhar, G. D., Academic Press, San Diego, 497–510, https://doi.org/10.1016/B978-0-08-091801-3.50041-6, 1993.
Liu, S., Chen, Y., Chen, Y., Friedman, J. M., Hati, J. H. A., and Fang, G.: Use of 2H and 18O stable isotopes to investigate water sources for different ages of Populus euphratica along the lower Heihe River, Ecol. Res., 30, 581–587, https://doi.org/10.1007/s11284-015-1270-6, 2015.
Liu, X., Silins, U., Lieffers, V. J., and Man, R.: Stem hydraulic properties and growth in lodgepole pine stands following thinning and sway treatment, Can. J. Forest Res., 33, 1295–1303, https://doi.org/10.1139/x03-061, 2011.
Maier, C. A., Burley, J., Cook, R., Ghezehei, S. B., Hazel, D. W., and Nichols, E. G.: Tree water use, water use efficiency, and carbon isotope discrimination in relation to growth potential in Populus deltoides and hybrids under field conditions, Forests, 10, 993, https://doi.org/10.3390/f10110993, 2019.
Manrique-Alba, À., Beguería, S., Molina, A. J., González-Sanchis, M., Tomàs-Burguera, M., del Campo, A. D., Colangelo, M., and Camarero, J. J.: Long-term thinning effects on tree growth, drought response and water use efficiency at two Aleppo pine plantations in Spain, Sci. Total Environ., 728, 138536, https://doi.org/10.1016/j.scitotenv.2020.138536, 2020.
Meinzer, F. C., Clearwater, M. J., and Goldstein, G.: Water transport in trees: current perspectives, new insights and some controversies, Environ. Exp. Bot., 45, 239–262, https://doi.org/10.1016/S0098-8472(01)00074-0, 2001.
Meinzer, F. C., Warren, J. M., and Brooks, J. R.: Species-specific partitioning of soil water resources in an old-growth Douglas-fir–western hemlock forest, Tree Physiol., 27, 871–880, https://doi.org/10.1093/treephys/27.6.871, 2007.
Meinzer, F. C., Woodruff, D. R., Eissenstat, D. M., Lin, H. S., Adams, T. S., and McCulloh, K. A.: Above- and belowground controls on water use by trees of different wood types in an eastern US deciduous forest, Tree Physiol., 33, 345–356, https://doi.org/10.1093/treephys/tpt012, 2013.
Molina, A., Vanacker, V., Balthazar, V., Mora, D., and Govers, G.: Complex land cover change, water and sediment yield in a degraded Andean environment, J. Hydrol., 472–473, 25–35, https://doi.org/10.1016/j.jhydrol.2012.09.012, 2012.
Moore, R. D. and Wondzell, S. M.: Physical Hydrology and the Effects of Forest Harvesting in the Pacific Northwest: A Review1, J. Am. Water Resour. Assoc., 41, 763–784, https://doi.org/10.1111/j.1752-1688.2005.tb03770.x, 2005.
Moore, R. D., Allen, D., MacKenzie, L., Spittlehouse, D., and Winkler, R.: Data sets for the Upper Penticton Creek watershed experiment: A paired-catchment study to support investigations of watershed response to forest dynamics and climatic variability in an inland snow-dominated region, Hydrol. Process., 35, e14391, https://doi.org/10.1002/hyp.14391, 2021.
Moreno-Gutiérrez, C., Barberá, G. G., Nicolás, E., De Luis, M., Castillo, V. M., Martínez-Fernández, F., and Querejeta, J. I.: Leaf δ18O of remaining trees is affected by thinning intensity in a semiarid pine forest, Plant Cell Environ., 34, 1009–1019, https://doi.org/10.1111/j.1365-3040.2011.02300.x, 2011.
Navarro-Cerrillo, R. M., Sánchez-Salguero, R., Rodriguez, C., Duque Lazo, J., Moreno-Rojas, J. M., Palacios-Rodriguez, G., and Camarero, J. J.: Is thinning an alternative when trees could die in response to drought? The case of planted Pinus nigra and P. Sylvestris stands in southern Spain, Forest Ecol. Manage., 433, 313–324, https://doi.org/10.1016/j.foreco.2018.11.006, 2019.
Ogle, K., Tucker, C., and Cable, J. M.: Beyond simple linear mixing models: Process-based isotope partitioning of ecological processes, Ecol. Appl., 24, 181–195, https://doi.org/10.1890/1051-0761-24.1.181, 2014.
Orlowski, N., Frede, H.-G., Brüggemann, N., and Breuer, L.: Validation and application of a cryogenic vacuum extraction system for soil and plant water extraction for isotope analysis, J. Sens. Sens. Syst., 2, 179–193, https://doi.org/10.5194/jsss-2-179-2013, 2013.
Park, J., Kim, T., Moon, M., Cho, S., Ryu, D., and Seok Kim, H.: Effects of thinning intensities on tree water use, growth, and resultant water use efficiency of 50-year-old Pinus koraiensis forest over four years, Forest Ecol. Manage., 408, 121–128, https://doi.org/10.1016/j.foreco.2017.09.031, 2018.
Pearcy, R. W., Ehleringer, J. R., Mooney, H., and Rundel, P. W.: Plant Physiological Ecology: Field methods and instrumentation, Springer Science & Business Media, 463 pp., ISBN 0412232308, 2012.
Prieto, I., Armas, C., and Pugnaire, F. I.: Water release through plant roots: new insights into its consequences at the plant and ecosystem level, New Phytol., 193, 830–841, 2012.
R Studio Team: R Studio: Integrated Development Environment for R, RStudio, PBC, Boston, MA U, http://www.rstudio.com/ (last access: 22 October 2024), 2020.
Russell, H. W.: Estimating Potential Evapotranspiration, Massachusetts Institude of Technology, 82 pp., http://hdl.handle.net/1721.1/79479 (last access: 22 October 2024), 1960.
Sánchez-Pérez, J. M., Lucot, E., Bariac, T., and Trémolières, M.: Water uptake by trees in a riparian hardwood forest (Rhine floodplain, France), Hydrol. Process., 22, 366–375, https://doi.org/10.1002/hyp.6604, 2008.
Simonin, K., Kolb, T. E., Montes-Helu, M., and Koch, G. W.: Restoration thinning and influence of tree size and leaf area to sapwood area ratio on water relations of Pinus ponderosa, Tree Physiol., 26, 493–503, https://doi.org/10.1093/treephys/26.4.493, 2006.
Simonin, K., Kolb, T. E., Montes-Helu, M., and Koch, G. W.: The influence of thinning on components of stand water balance in a ponderosa pine forest stand during and after extreme drought, Agr. Forest Meteorol., 143, 266–276, https://doi.org/10.1016/j.agrformet.2007.01.003, 2007.
Sohn, J. A., Kohler, M., Gessler, A., and Bauhus, J.: Interactions of thinning and stem height on the drought response of radial stem growth and isotopic composition of Norway spruce (Picea abies), Tree Physiol., 32, 1199–1213, https://doi.org/10.1093/treephys/tps077, 2012.
Sohn, J. A., Brooks, J. R., Bauhus, J., Kohler, M., Kolb, T. E., and McDowell, N. G.: Unthinned slow-growing ponderosa pine (Pinus ponderosa) trees contain muted isotopic signals in tree rings as compared to thinned trees, Trees, 28, 1035–1051, https://doi.org/10.1007/s00468-014-1016-z, 2014.
Sohn, J. A., Saha, S., and Bauhus, J.: Potential of forest thinning to mitigate drought stress: A meta-analysis, Forest Ecology and Management, 380, 261–273, https://doi.org/10.1016/j.foreco.2016.07.046, 2016.
Sprenger, M., Tetzlaff, D., and Soulsby, C.: Soil water stable isotopes reveal evaporation dynamics at the soil–plant–atmosphere interface of the critical zone, Hydrol. Earth Syst. Sci. 21, 3839–3858, https://doi.org/10.5194/hess-21-3839-2017, 2017.
Stagge, J. H., Tallaksen, L. M., Xu, C. Y., and Lanen, H. A. J. V.: Standardized precipitation-evapotranspiration index (SPEI): Sensitivity to potential evapotranspiration model and parameters, Hydrology in a Changing World, in: 7th World Flow Regimes from International and Experimental Network Data-Water Conference, FRIEND-Water 2014, 7–10 October 2014, Montpellier, France, 367–373, ISBN 9781907161414, 2014.
Stock, B. C. and Semmens, B. X.: MixSIAR GUI User Manual, Version 3.1, Zenodo [code], https://doi.org/10.5281/zenodo.1209993, 2016.
Stock, B. C., Jackson, A. L., Ward, E. J., Parnell, A. C., Phillips, D. L., and Semmens, B. X.: Analyzing mixing systems using a new generation of Bayesian tracer mixing models, Peer J., 6, e5096, https://doi.org/10.7717/peerj.5096, 2018.
Streck, N. A.: Stomatal Response to water vapor pressure deficit: An unsolved issue, Curr. Agricult. Sci. Technol., 6, 317–322, 2003.
Stumpp, C., Brüggemann, N., and Wingate, L.: Stable Isotope Approaches in Vadose Zone Research, Vadose Zone J., 17, 180096, https://doi.org/10.2136/vzj2018.05.0096, 2018.
Szymczak, S., Barth, J., Bendix, J., Huneau, F., Garel, E., Häusser, M., Juhlke, T., Knerr, I., Santoni, S., Mayr, C., Trachte, K., van Geldern, R., and Bräuning, A.: First indications of seasonal and spatial variations of water sources in pine trees along an elevation gradient in a Mediterranean ecosystem derived from δ18O, Chem. Geol., 549, 119695, https://doi.org/10.1016/j.chemgeo.2020.119695, 2020.
Tsuruta, K., Yamamoto, H., Katsuyama, M., Kosugi, Y., Okumura, M., and Matsuo, N.: Effects of cryogenic vacuum distillation on the stable isotope ratios of soil water, Hydrol. Res. Lett., 13, 1–6, https://doi.org/10.3178/hrl.13.1, 2019.
Vargas, A. I., Schaffer, B., Yuhong, L., and Sternberg, L. da S. L.: Testing plant use of mobile vs immobile soil water sources using stable isotope experiments, New Phytol., 215, 582–594, 2017.
Wang, J., Fu, B., Lu, N., and Zhang, L.: Seasonal variation in water uptake patterns of three plant species based on stable isotopes in the semi-arid Loess Plateau, Sci. Total Environ., 609, 27–37, https://doi.org/10.1016/j.scitotenv.2017.07.133, 2017.
Wang, T., Xu, Q., Gao, D., Zhang, B., Zuo, H., and Jiang, J.: Effects of thinning and understory removal on the soil water-holding capacity in Pinus massoniana plantations, Sci. Rep., 11, 13029, https://doi.org/10.1038/s41598-021-92423-5, 2021.
Wang, Y., Wei, X., del Campo, A. D., Winkler, R., Wu, J., Li, Q., and Liu, W.: Juvenile thinning can effectively mitigate the effects of drought on tree growth and water consumption in a young Pinus contorta stand in the interior of British Columbia, Canada, Forest Ecol. Manage., 454, 117667, https://doi.org/10.1016/j.foreco.2019.117667, 2019.
Warren, J. M., Meinzer, F. C., Brooks, J. R., and Domec, J. C.: Vertical stratification of soil water storage and release dynamics in Pacific Northwest coniferous forests, Agr. Forest Meteorol., 130, 39–58, https://doi.org/10.1016/j.agrformet.2005.01.004, 2005.
Wassenaar, L. I., Athanasopoulos, P., and Hendry, M. J.: Isotope hydrology of precipitation, surface and ground waters in the Okanagan Valley, British Columbia, Canada, J. Hydrol., 411, 37–48, https://doi.org/10.1016/j.jhydrol.2011.09.032, 2011.
West, A. G., Patrickson, S. J., and Ehleringer, J. R.: Water extraction times for plant and soil materials used in stable isotope analysis, Rapid Commun. Mass Spectrom., 20, 1317–1321, https://doi.org/10.1002/rcm.2456, 2006.
Winkler, R., Diana, A., Giles, T., Heise, B., Moore, R. D., Redding, T., Spittlehouse, D., and Wei, X.: Approaching four decades of Forest Watershed research at Upper Penticton Creek, British Columbia: A Synthesis, Hydrol. Process., 35, e14123, https://doi.org/10.1002/hyp.14123, 2021.
Winkler, R. D. and Moore, R. D.: Variability in snow accumulation patterns within forest stands on the interior plateau of British Columbia, Canada, Hydrol. Process., 20, 3683–3695, https://doi.org/10.1002/hyp.6382, 2006.
Wu, H., Hayes, M. J., Wilhite, D. A., and Svoboda, M. D.: The effect of the length of record on the standardized precipitation index calculation, Int. J. Climatol., 25, 505–520, https://doi.org/10.1002/joc.1142, 2005.