the Creative Commons Attribution 4.0 License.
the Creative Commons Attribution 4.0 License.
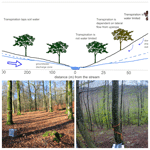
The influence of hillslope topography on beech water use: a comparative study in two different climates
Ginevra Fabiani
Julian Klaus
Daniele Penna
Understanding the interrelation between topography and vegetation across different environments is important to assess how hydrological and climatic conditions affect tree physiological activity. This becomes especially important given the expected reduction in water availability and the increase in water demand driven by climate change. These extremes could enhance the thermal and hydrologic gradients along slopes. Here, we aimed to test if and how different climatic and hydrological conditions affect the physiological response of beech trees (Fagus sylvatica L.) to environmental variables along two different topographic sequences. For this purpose, we set up a comparative study on a gentle hillslope in the Weierbach catchment in Luxembourg (oceanic climate) and on a steep hillslope in the Lecciona catchment in Italy (Mediterranean climate). We combined sap velocity measurements with isotopic measurements of soil, precipitation, stream water, groundwater, and xylem over 2019 and 2020 for the Luxembourgish site and over 2021 for the Italian site. We found that, in the Weierbach catchment, trees' responses to environmental variables (i.e. vapour pressure deficit and relative extractable water in the soil) were similar among hillslope positions and between the two monitored years, resulting from homogeneous growing conditions along the topographic sequence. We also did not find any statistical difference in the isotopic composition of xylem water between positions, suggesting that beech trees relied on similar water sources across the landscape. In the Lecciona catchment, we observed lower sap velocities and shorter growing season in trees growing in the upper portions of the hillslope, likely related to water redistribution and different soil moisture along the hillslope catena. Xylem isotopic composition was significantly lighter at the footslope location throughout the growing season than in the upslope locations, suggesting location-specific water use. These results emphasize how differing hydrometeorological processes occurring at the hillslope scale can lead to contrasting tree responses.
- Article
(8689 KB) - Full-text XML
- BibTeX
- EndNote
Global change is strongly affecting forest ecosystems by exacerbating environmental extremes, such as droughts and heatwaves (Allen et al., 2010; Choat et al., 2012). The increasing atmospheric evaporative demand (i.e. vapour pressure deficit; VPD) and reduced water availability (i.e. relative extractable water; REW) in soils expose trees to water deficit risk (Salomón et al., 2022), impairing tree functioning (Giménez et al., 2013). Water availability is variable in space and time and among domains of the hydrological cycle (i.e. atmosphere, belowground, surface water) (Klaus et al., 2022; Tijdeman et al., 2022), and changes in water availability are expected to affect the distribution of tree species based on their ecophysiological adaptations to varying water conditions (Hahm et al., 2018). Multiple studies on the effect of reduced water availability on forest vulnerability have neglected plot-scale landscape heterogeneity, even though soil properties and topography are important abiotic factors for forest health (Schwantes et al., 2018). The interrelation of these factors results in thermal and water gradients across landscapes and ultimately shapes species composition and distribution (Méndez-Toribio et al., 2017). Belowground properties determine the water flow patterns along a hillslope (Penna et al., 2015; Klaus and Jackson, 2018; Xiao et al., 2021), resulting in different water availabilities in the subsurface, one of the main drivers of tree productivity (Hogg et al., 2008). Compared to uphill locations, topographically convergent areas are generally wetter due to the lateral redistribution of water and increasing contributing area (Jencso et al., 2009; Western et al., 1999; Penna et al., 2009). The steepness of the slope leads to a spatially variable microclimate, which is generally less evaporatively demanding at the footslope, where wind speed and solar radiation are reduced (Hoylman et al., 2018). Deeper soils with higher storage capacity and organic matter typically occur at the footslope and are associated with greater stand primary productivity compared to hilltop locations (Elliott et al., 2015; Hawthorne and Miniat, 2018; Tromp-van Meerveld and McDonnell, 2006), where trees may experience more water stress (Oberhuber and Kofler, 2000). The effects of the physiological drought, which occurs when water is not sufficient to sustain plant needs, are dampened in locations where soil water storage capacity is higher (Meusburger et al., 2022). Spatially variable soil characteristics and depths can result in variable degrees of water stress for the same forest species at the same site (Tai et al., 2017). With the expected increase in the frequency and intensity of drought events, topography-related changes in soil moisture availability (Hawthorne and Miniat, 2018) and a changing land surface energy balance (Dobrowski, 2011) may lead to an increasing gradient of water stress in the vegetation from the lower to the higher parts of the hillslope catena. During dry periods, topographic factors may amplify the response of transpiration to soil water limitation (Renner et al., 2016). Greater water availability in concave areas does not necessarily translate into high tree growth because persistently saturated conditions in the subsurface restrict root expansion to the vadose zone above the water table (Fan et al., 2017). Bosch et al. (2014) found that landscape position – and, subsequently, access to groundwater – did not appear to strongly influence sap flow rates. Instead, sap flow rates were mainly influenced by water content in the vadose zone (Bosch et al., 2014). Similarly, Fabiani et al. (2022b) found that a high, steady groundwater table does not necessarily enhance sap velocity in two co-occurring species in a temperate forest. Low oxygen levels in saturated soil or regolith can hamper root functioning and development in trees sensitive to anaerobic conditions and can force them to rely on shallow soil water and use little groundwater (Thorburn et al., 1993). Interannual meteorological variability represents another potentially important control on the patterns of plant physiological activity (Murphy et al., 2020). Vegetation can deploy physiological (i.e. stomatal conductance) and structural (i.e. leaf area, crown architecture, rooting depth) adaptations to acclimate to changing hydrometeorological conditions at annual to decadal timescales (Grossiord et al., 2017a; McDowell et al., 2008).
European beech (Fagus sylvatica L.) is among those species that do not grow roots in the saturated zone and that mainly rely on water stored in the shallow soil (Gessler, 2021; Kreuzwieser and Rennenberg, 2014). European beech is a key component of the European mesic forests, with a distribution range covering wide climatic and geological settings (Houston Durrant et al., 2016). In the last decade, its physiological activity (i.e. leaf phenology and cambial activity) has been mildly to severely restricted by droughts in 2003, 2015, and 2018 (Cavin and Jump, 2017; Obladen et al., 2021; Salomón et al., 2022; Walthert et al., 2021). It is expected that European beech will face high mortality rates with the predicted increase in heatwaves. The hydraulic system of beech trees can show pronounced modifications in response to drought (Zimmermann et al., 2021), but it is still unclear how beech trees will cope with a future increase in VPD and REW. So far, site-specific case studies with contrasting results have offered a limited understanding of how hillslope-scale processes affect tree water use in complex terrain. This information cannot be retrieved from larger-scale approaches (Murphy et al., 2020). Therefore, detailed hillslope-scale field studies are needed to identify where and when tree physiological activity is more constrained by increasing VPD and REW. This can support the development of management practices suitable for locations at risk. So far, ecohydrological studies have predominantly concentrated on variations in water use between species, with limited attention given to within-species dynamics (Li and Knighton, 2023), constraining our ability to understand how diverse climates influence the physiological responses of a species. Through a cross-site comparison, we aim to advance our knowledge of the water use strategies of beech trees under different climatic and topographic conditions in order to allow for a better understanding of the ecohydrological functioning of forest ecosystems. For this reason, we set up a comparative study on a gentle hillslope in the Weierbach catchment in Luxembourg (oceanic climate) and on a steep hillslope in the Lecciona catchment in Italy (Mediterranean climate). These sites differed in terms of steepness, climate, geology, and soil characteristics, but beech is the dominant species in both. We combined sap velocity and isotope measures with environmental monitoring to capture beech trees' water response to environmental variables along hillslope transects. While sap velocity measurements allow a continuous assessment of the trees' physiologic responses to environmental drivers, stable isotopes of xylem water offer insights into the origin of the water utilized. We hypothesize that (i) the physiological response of beech trees varies as a function of topography-driven changes in water availability, and (ii) at the Mediterranean site, characterized by an uneven precipitation regime and high summer evaporative demand, the physiological activity of beech trees is more constrained compared to the stand growing in the Luxembourgish site. We test these hypotheses by posing the following research questions: (i) does topography drive different water uptake strategies along two contrasting hillslopes and (ii) does hillslope topography increase tree sensitivity to a seasonal decrease in soil water supply?
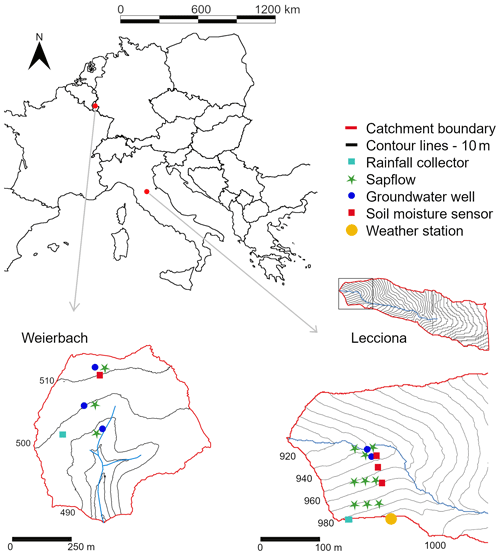
Figure 1Site overview with sensors in place: on the left is the Weierbach catchment in Luxembourg, and on the right is the Lecciona catchment in Tuscany, Italy. In the Weierbach, we monitored groundwater level (blue dot) and sap velocity (green star) in one beech tree at each hillslope position and soil moisture (red square) at the plateau. In the Lecciona catchment, we measured sap velocity (green star) in three trees and soil moisture content (red square) at the midslope, footslope location, and riparian area, and groundwater level (blue dot) was recorded at the footslope and in the riparian area.
2.1 Study sites
2.1.1 The Weierbach catchment: study area and environmental monitoring
The experiment was carried out during two consecutive growing seasons (March–October 2019 and 2020) at a transect along a south-facing forested hillslope ranging from 485 to 515 in the Weierbach catchment (42 ha) in Luxembourg (Fig. 1) (Hissler et al., 2021; Pfister et al., 2023). The site description and characterization of the plateau, midslope, and footslope locations have been provided in detail by Fabiani et al. (2022b). Here, we report only the key details of the study site (Table 1). The hillslope transect is 380 m long and spans from the stream bank to a plateau area with an average slope of 10°. Based on the results of a forest survey conducted at the study site, the forest consists of European beech trees (Fagus sylvatica L.) (78% of trees, 60% in basal area) and pedunculate and sessile oak hybrid trees (22% of trees, 40% in basal area). The moderate, oceanic climate in the region is largely influenced by air masses from the northern Atlantic Ocean (Hissler et al., 2021). Long-term annual precipitation (2007–2020) is approximately 801 mm at the Roodt weather station (3.5 km from the study site), of which 452 mm falls during the growing season (1 April–31 October). The mean annual air temperature during the growing season from 2007 to 2020 was 8.3 °C. Volumetric soil moisture was measured at the plateau at 10, 20, 40, and 60 cm depth with water content reflectometers (CS650, Campbell Scientific, UK). Groundwater wells were installed along the hillslope transect at the plateau, midslope, and footslope locations. Groundwater table depth was monitored with water pressure transducers (Orpheus Mini, OTT, Germany) recording data at 15 min logging intervals.
2.1.2 The Lecciona catchment: study area and environmental monitoring
Lecciona (Fig. 1) is a subcatchment (31 ha) of the Re della Pietra experimental catchment (2 km2) located in the Apennines in Tuscany, central Italy. We selected a north-facing hillslope transect ranging from 940 to 970 with an average slope of 30° (Table 1). The selected hillslope spanned from the stream to the highest point of each catchment to address the extreme locations and the progressive edaphic and environmental changes. We defined the hilltop as the highest point in the slope (close to the catchment ridgeline), the footslope location as being in the proximity of the stream, and the midslope location as being situated between the two. The hillslope is covered by a pure stand of beech trees with sparse oak individuals at the ridge top. Understorey and tree regeneration are absent, and a thick litter layer of leaves washed off the upper portion of the slope accumulates downslope. At the ridgeline, trees are replaced by Scotch broom bushes (Cytisus scoparius L.), a species that typically grows in dry and sunny sites in secondary succession stages. The climate in the study area is typical of Mediterranean mountain environments, with long-term annual precipitation (2001–2021) of 1265 mm, of which 590 mm falls during the growing season (April–October), as recorded at the Consuma weather station (ca. 10 km from the study site, managed by the regional meteorological service). The meteorological station at the study site was installed in late 2018 and recorded a mean air temperature of 15.1 °C during the growing season (1 April–31 October) over 2018–2021. The lithology consists of Macigno sandstone, a turbidite unit with pelitic-dominant lithofacies and a reduced thickness. Soil texture was assessed on 11 samples distributed along the hillslope. Sand content ranged between 57% and 76%, and clay content ranged between 4% and 11%. Soil texture in all samples resulted in sandy loam, according to the USDA classification. Volumetric soil moisture was measured at the midslope, footslope, and riparian area at 15 and 35 cm depth with water content reflectometers (TEROS 10, METER Group). The standard calibration for organic soils recommended by the manufacturer was applied to the soil moisture probes. As the analyses in this work focused on the dynamics of soil moisture and not on the absolute values of soil moisture, the lack of a specific soil calibration did not affect the data interpretation and the results. Groundwater wells were drilled in 2019 at the footslope and in the riparian area. Wells were equipped with water pressure transducers (Orpheus Mini, OTT, Germany) recording data at 15 min logging intervals. Meteorological data (precipitation, air temperature, air relative humidity, wind speed, and wind direction and radiation) were monitored at 5 min resolution in an open area at the top of the hillslope at 991
Based on long-term records, the Lecciona catchment consistently receives more precipitation than the Weierbach catchment, both annually and throughout the growing season. While precipitation is evenly distributed across seasons in the Weierbach catchment, it falls mostly during the dormant season in the Lecciona catchment, which is typical of a Mediterranean climate (data not shown).
2.2 Relative extractable water (REW) and vapour pressure deficit (VPD)
In order to account for inter-site differences in soil properties and to facilitate the inter-site comparison, we estimated the daily relative extractable water (REW, unitless) (Granier et al., 1999) over all monitored depth classes at each site, calculated as follows:
where SM is the daily soil moisture obtained by averaging data from all the sensors, and SM95th and SMmin are the site-specific soil moisture approaching field capacity and minimum values, respectively. REW varies between 0 and 1 and represents the ratio between available soil water and maximum extractable water. REW was calculated for the Weierbach catchment based on soil moisture records from 2012 to 2020 and for the Lecciona catchment from 2020 to 2021. Following Salomón et al. (2022), we chose the 95th percentile and not the maximum value to exclude heavy-rain events that may result in soil saturation. REW has been quantified in previous studies to characterize soil drought intensity (Bréda et al., 2006; Grossiord et al., 2017b) and to carry out inter-site comparison (Salomón et al., 2022; Granier et al., 2007).
For each study site, we computed the daily mean vapour pressure deficit (VPD, kPa), calculated as follows:
where T and RH are the air temperature and relative air humidity recorded by the weather station at each study site (Fig. 1).
2.3 Measurement of sap velocity
At each site, we defined three monitoring locations corresponding to different topographic positions: the plateau, midslope, and footslope in the Weierbach catchment and the hilltop, midslope, and footslope in the Lecciona catchment (Fig. 1). For each monitoring location, we selected one tree in the Weierbach catchment and three trees in the Lecciona catchment (Fig. 1). By evaluating the forest stand characteristics and the crown structure, we selected mature trees with approximately the same phytosociological positions and diameters in each site to exclude tree-size-related effects (diameters between 30 and 32 cm at both sites). The selected trees were equipped with heat pulse sap flow sensors (SFM1, ICT International Pty Ltd., Australia), which record sap velocity at distances of 12.5 and 27.5 mm from the bark. The sensors were installed before leaves flushed and remained in place until leaves had fallen. In 2020, the installation in the Weierbach catchment was delayed due to COVID-19 restrictions that prevented field work. In the Weierbach catchment, we positioned the sap flow sensors on the northeastern side of the trunk at 1.3 m height. In the Lecciona catchment, we opted to install them on the west side to avoid placement in tension wood, which is characterized by lower lignification and eccentric growth in hardwood species on steep slopes. The heat pulse technique uses heat as a tracer to determine sap velocity. Briefly, a heater and two temperature sensors are inserted into holes drilled horizontally into the sapwood, with the sensors at a fixed distance above and below the heater (5 mm). More details on the method can be found in Burgess et al. (2001). The mechanical damage of sensor installation induces xylem wounding, interrupting the water flow path (Barrett et al., 1995) and causing an increase in the proportion of non-conducting tissue. In order to account for this effect, we applied a wound correction factor of 0.13 cm (Green et al., 2003). We averaged sap velocities measured on an hourly basis by the inner and outer thermistor, and then we calculated a daily mean sap velocity, which is used as a proxy for tree transpiration (Smith and Allen, 1996). We filled the data gaps due to power malfunctioning via linear interpolation with sensors at the same location (Table A1 and Fig. A1 in the Appendix).
2.4 Wood core, soil, and water sampling
We sampled tree xylem water for analysis of its isotopic composition biweekly over the 2019 and 2020 growing seasons in the Weierbach catchment (26 sampling campaigns) and in 2021 in the Lecciona catchment (11 sampling campaigns). The trees selected for xylem sampling were in the same diameter range as the trees equipped with sap flow sensors and were in close proximity to each other (within 25 m). Tree cores encompassing only the sapwood (ca. the outer 3.5 cm of xylem) were collected around the stem circumference with a Pressler borer. We avoided sampling above or below previous cores to minimize possible disturbances in sampled trees due to prior wounding. Xylem samples were quickly placed in glass vials, capped, and sealed with Parafilm©. We simultaneously sampled soil cores to determine the soil water isotopic composition. At each sampling area, we extracted three soil cores from the uppermost 60 cm with a soil auger and divided them into five depth classes (0–5, 5–10, 10–20, 20–40, and 40–60 cm). Soil samples were collected in laminated aluminium bags, which have proven to be effective in minimizing evaporation even several days after the soil sample collection (40 d) (Gralher et al., 2021), and we heat-sealed all bags upon arrival at the laboratory at the Luxembourg Institute of Science and Technology (LIST). The Weierbach soil samples were processed and analysed upon arrival at the laboratory. More details are reported in Fabiani et al. (2022b). Lecciona soil samples were kept frozen at −10° and were shipped to the laboratory at the end of the growing season of 2021, and they were kept frozen until analysis. The freezing process prior to analysis reduces the microbial activity and the associated buildup of CO2 (Gralher et al., 2021). We sampled groundwater at the sampling areas in the Weierbach catchment, and we also sampled rainfall biweekly with an evaporation-protected rainfall collector (Palmex Ltd.) placed in a clearing at both sites (Fig. 1). In the Lecciona catchment, groundwater was sampled on two occasions in 2022 (24 March and 5 October) due to logistical difficulties.
2.5 Laboratory analyses
Xylem water was extracted via a cryogenic water extraction line (Orlowski et al., 2016) at LIST. Briefly, samples were submerged in a 100 °C oil bath for 3 h under a vacuum (0.03 hPa), and the resulting water vapour was collected in U-shaped tubes placed in liquid nitrogen (−197 °C). We assessed the gravimetric water content for xylem samples by weighing them before and after cryogenic water extraction. We quantified the wood moisture content (%) as the ratio of the weight of extracted water (liquid mass) to the weight of dry wood after cryogenic water extraction (Peck, 1953; Steppe et al., 2010; Fabiani et al., 2022a). The isotopic composition (δ2H and δ18O) of the extracted water was analysed using a Picarro cavity ring-down spectrometer (CRDS) (L2140-i, Picarro Inc., Santa Clara, CA, United States) coupled with a Micro-Combustion Module™ (MCM) to remove organic compounds (ethanol, methanol, and/or other biogenic volatile compounds). To detect possible organic contamination, xylem samples were screened with ChemCorrectTM (Picarro Inc., Santa Clara, CA, United States) software, which attempts to identify contamination by fitting to a known library of spectral features. No organic contamination was found in any sample. To minimize the memory effect, each sample was analysed 10 times, and only the last five readings were saved and averaged (Penna et al., 2012). We analysed the isotopic composition of soil samples with the direct liquid water–vapour equilibration method (Wassenaar et al., 2008). We let the samples thaw for 2 d while equipping the aluminium bags with silicon blots. A total time of 48 h was sufficient to ensure the hardening of the silicone and to minimize the risk of outgassing volatile organic compounds from freshly applied silicone (Gralher et al., 2021). We inflated the bags with dry air by puncturing the hardened silicon blots, and we let them equilibrate for 48 h at a constant temperature for isothermal equilibration. Headspace vapour was sampled directly with a needle connected to the Picarro analyser coupled with the MCM for the samples and the five bags with known isotopic composition (ranging between −16.2 to −2.9 in terms of δ18O and −123.7 to −9.9 in terms of δ2H). Water vapour concentrations were always above 28 000 ppm where the concentration-dependent deviation became low, and thus measurement precision was not compromised. The local meteoric water line (LMWL) at the Weierbach catchment is δ2H = 7.4δ18O+7.4 and is based on biweekly precipitation samples collected from 2011 to 2020. This rather low slope is characteristic of the region (Klaus et al., 2015). The LMWL at the Lecciona catchment is δ2H = 6.2δ18O+7.0 and is based on biweekly to monthly samples of precipitation collected from 2018 to 2021.
2.6 Data analysis
We applied linear mixed-effect models (LMEMs) with the lme4 package (Bates et al., 2015) to determine the combined effect of environmental variables (VPD, REW, and radiation W) and topographic positions on sap velocity over the course of the growing season. We fitted an LMEM for each study site to identify the most important predictors of monthly transpiration. Sap velocity was used as dependent variable, with VPD, W, and REW being environmental predictors. Individual trees were used as the random-effect term included in all models. Furthermore, since we were interested in the response of sap velocity to environmental conditions (i.e. REW and VPD) as an indicator of stomatal control, we normalized daily mean sap velocities between 0 and 1, where 0 and 1 were the minimum and maximum daily mean velocities recorded by each tree over the entire growing season. This procedure facilitates the comparison between the two study sites, as shown elsewhere (Gimenez et al., 2019). Comparisons of sap velocities between locations were analysed with the pairwise Wilcox test. The pairwise Wilcox test was also used to analyse differences in the isotopic composition of groundwater sampled at the three sampling areas. Comparisons of the xylem isotopic composition between locations were analysed via the non-parametric Mann–Whitney test. The significance level was set to α ≤ 0.05 to determine which years or locations were statistically different from each other. For all our analyses, we used R software version v.4.2.0 (R Core Team, 2021).
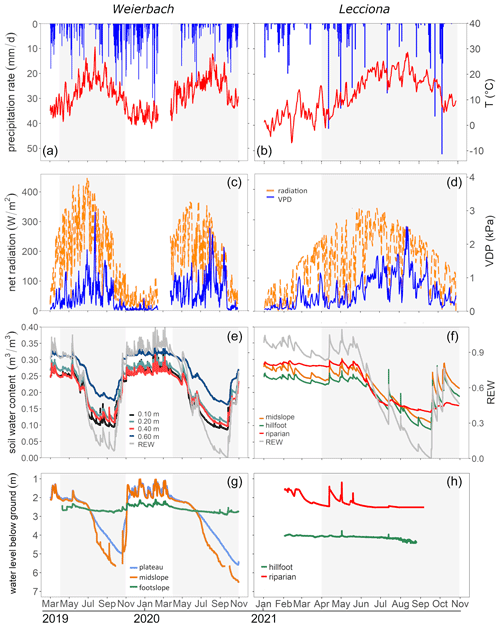
Figure 2Daily total precipitation amount (mm d−1), daily mean air temperature (°C), daily mean radiation (W m−2), and daily mean vapour pressure deficit (VPD) (kPa) observed at the Roodt (left plots, a and c) weather station and the Lecciona catchment (right plots, b and d). Volumetric soil water content (m3 m−3) and REW (relative extractable water) in the Weierbach catchment (e) and the Lecciona catchment (f). Soil moisture in the Weierbach catchment was measured at four depths in the plateau location. In the Lecciona catchment, soil moisture was recorded in three topographic positions at two depths, which are averaged in the plot. Groundwater level below ground surface (m) in the Weierbach catchment (g) and the Lecciona catchment (h). The shaded area represents the growing season.
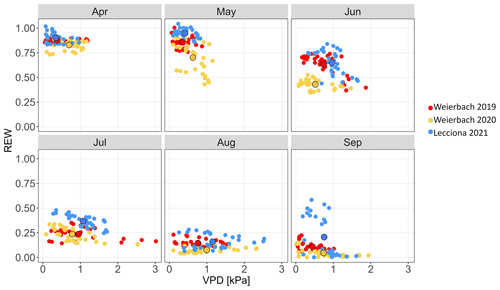
Figure 3Relationship between VPD (kPa) and REW over the growing season (2019–2020) in the Weierbach catchment and (2021) in the Lecciona catchment. Filled dots with black outlines represent monthly means.
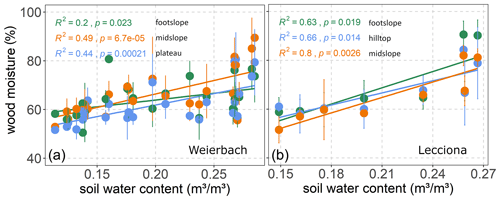
Figure 4Relationship between wood moisture content (%) and volumetric soil moisture content of the xylem sampling date in the Weierbach catchment (a) and the Lecciona catchment (b). Solid dots represent the mean of xylem moisture, and the whiskers indicate the confidence interval (95%). In the Weierbach catchment, we used the average soil moisture content over the four depths, while in the Lecciona catchment, we used the average soil moisture over the two monitored depths and the three locations.
3.1 Environmental conditions across years and sites
In the Weierbach catchment, the 2020 growing season was characterized by drier conditions compared to 2019 and precipitation depth was below the long-term average (2007–2019) of 451 mm (Schoppach et al., 2021). Despite the lower average solar radiation in 2020 than in 2019 (Table 2, Fig. 2c), the 2020 growing season was characterized by higher evaporative demand concurrently with reduced precipitation input (Table 2, Fig. 2a) and lower soil moisture (Table 2, Fig. 2e) compared to 2019. The lowest REW value within the period 2002–2020 was reached in 2020 in the second half of September (data not shown). However, the daily maximum VPD was higher in 2019 with 3 kPa compared to 2020 when VPD reached 2.5 kPa. In the hydrometeorological space, defined by the relationship between REW and VPD (Salomón et al., 2022), each month of the 2020 growing season was characterized by lower REW but higher VPD than in 2019, except for June and July, when VPD was higher in 2019 (Fig. 3). This general dry-out of the system was also observed in the groundwater table that progressively declined (Fig. 2g) up to 5.58 and 6.50 m in depth in 2020 compared to 4.98 and 5.65 m in 2019 in the plateau and midslope, respectively. The maximum and minimum groundwater table depth did not vary between the 2 years at the footslope location (Fig. 2g). The decline in soil moisture was accompanied by a progressive dehydration of xylem (Fig. A3), leading to a significant relationship between soil moisture and wood moisture content (Fig. 4). In the Weierbach catchment, there were no statistical differences in wood water content between the 2019 and 2020 growing seasons or when the values of each month were compared (Kruskal–Wallis, p > 0.05). However, wood moisture was significantly lower in trees located at the plateau (Kruskal–Wallis, p = 0.04) compared to trees growing in the other locations.
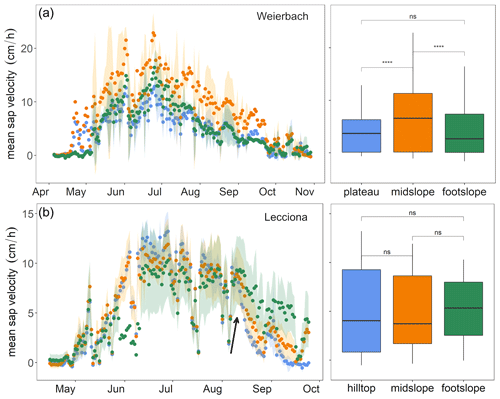
Figure 5Daily mean sap velocity (cm h−1) of Weierbach (a) and Lecciona (b) trees. In this figure, the Weierbach catchment data were averaged between the 2019 and 2020 growing seasons, while data from the Lecciona catchment cover the 2021 growing season. Each colour corresponds to location (green indicates the footslope, orange indicates the midslope, and blue indicates the hilltop or plateau). The area inside the coloured lines is the mean average deviation among trees at the same location for the Lecciona catchment and between monitored years in the Weierbach catchment. Boxplots on the right side show the average sap velocity by location. The whiskers indicate points within 1.5 times the interquartile range above or below the median. Above each boxplot, asterisks (symbols “∗” and “ns” correspond to p values ≤ 0.05 or > 0.05, respectively) denote significant statistical differences between locations (Wilcoxon test). The black arrow in panel (b) indicates the sudden drop of mean sap velocity in trees of the hilltop position in the Lecciona catchment.
In the Lecciona catchment, the 2021 growing season was characterized by a higher average temperature compared to the Weierbach catchment (Table 2) but with a similar precipitation amount. Soil moisture content was, on average, similar between the two sites (Table 2). In the Lecciona catchment, soil moisture decreased from June until September, when precipitation events replenished the soil water content. Soil moisture at the midslope and footslope decreased simultaneously, while soil moisture in the riparian area decreased to a lesser extent and remained higher and steadier (Fig. 2f). The groundwater table at the footslope location was rather constant, with an average depth of 4.1 m, and responded only after major rainfall events (Fig. 2h). There was no statistical difference in wood moisture content between locations in the Lecciona catchment (Kruskal–Wallis, p = 0.72). By comparing the hydrometeorological space between the two study sites, we found slightly higher REW and higher VPD in the Lecciona catchment compared to in the Weierbach catchment (Fig. 3). The progressive decrease in precipitation input over the growing season affected not only soil moisture but also the wood water content (Fig. A3). There was a significant relationship between soil moisture and wood moisture content at all locations (Fig. 4) and a marked temporal variability (Fig. A3). According to hydrometeorological space (Fig. 3), we selected the months with more contrasting environmental conditions (i.e. June, August, September) in terms of water availability relative to the maximum extractable water (REW) and evaporative demand (VPD). To investigate the tree physiological response to the site-specific environmental stress and to compare between locations, we plotted the normalized sap velocity against sites' daily VPD and REW for the selected months (Fig. 3).
3.2 Sap velocity
In the Weierbach catchment, there was a consistent pattern in sap velocity between the consecutive monitoring years, with monthly sap velocities being higher in June compared to other months (data not shown) but with daily sap velocity peaking in July (Fig. 5). Sap velocity was significantly higher (Kruskal–Wallis test, p < 0.001) at the midslope location than at other locations, followed by the plateau and footslope when both years were considered (Fig. 5). Sap velocities were statistically higher in 2020 than in 2019 in May and September at all locations. Despite the early yellowing and shedding of leaves observed in August 2020, which are symptoms of partial dysfunction of the hydraulic system, sap velocities were not lower than in 2019.
In the Lecciona catchment, there were no statistical differences in the overall mean velocity among locations (Wilcoxon test, p > 0.3) (Fig. 5b). However, there was a location-specific behaviour by month. All trees shared a common pattern for the first part of the growing season: after leaf-out (19–26 April), sap velocity increased for all trees and locations and peaked toward the end of June (Fig. 5). From August onwards, sap velocity markedly dropped in plants at the hilltop (Fig. 5; see arrow). In contrast, sap velocity remained high and did not vary between July and August at the footslope. Trees at the midslope location displayed behaviour that was in between that of the other locations. In September, the sap velocity of trees located at the footslope was significantly higher than that at the other locations (Kruskal–Wallis, p < 0.05) and was 2- and 4-fold higher compared to the midslope and hilltop, respectively. The growing season (leaf-out) started later in the Lecciona catchment compared to the Weierbach catchment; data are not available from the late season (October onwards) to compare the duration of the growing season between the two sites.
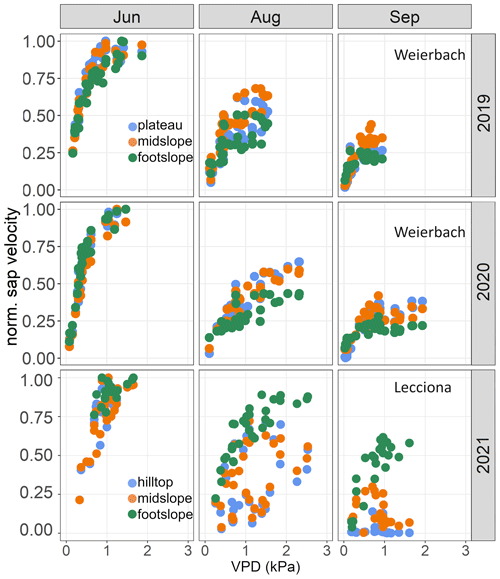
Figure 6Relationship between normalized mean sap velocity and daily vapour pressure deficit (VPD) (kPa) at different hillslope positions in June, August, and September in 2019 and 2020 in the Weierbach catchment and in 2021 in the Lecciona catchment.
In the Weierbach catchment, sap velocity was always positively correlated with an increase in VPD (p < 0.01) and W (p < 0.01) from June onwards (Fig. A2). High REW values were followed by a decrease in sap velocity (p < 0.01) only in the early phases of the growing season and later on by an increase in sap velocity. LMEM analyses showed that hillslope position did not explain sap velocity variation in either 2019 or 2020. In the Lecciona catchment, VPD and W were the best predictors explaining an increase in sap velocity (p < 0.01). However, LMEMs showed that hillslope position (p < 0.01) and W (p < 0.01) explained most of the variability in sap velocity in September (Fig. A2).
3.3 Sap velocity response to VPD and REW
The relationship between normalized sap velocity and VPD in the growing season did not differ between locations and years in the Weierbach catchment (Fig. 6). However, for the same VPD values, sap velocity was lower in August and September at the footslope compared to at the other locations, indicating a stronger stomatal control on transpiration fluxes. There was a progressive decline in sap velocity throughout the growing season despite similar monthly VPD (Fig. 3). In both years, maximum sap velocities were reached when VPD ranged between 1.0 and 1.4 kPa (data not shown). In the Lecciona catchment, there was a strong differentiation in terms of sap velocity response to VPD across the topographic sequence (Fig. 6). For the first months of the growing season, sap velocity response to VPD was consistent among hillslope positions. In August, trees at the footslope maintained a high sap velocity even when daily mean VPD values were 2.7 kPa, the highest value of the season (Fig. 2d). On the other hand, sap velocity of trees at the hilltop and midslope declined progressively in August and ceased in September while remaining high at the footslope. There was no difference in the relationship between normalized sap velocity and REW among hillslope positions and over the years in the Weierbach catchment (Fig. 7). Following the reduction in REW over the months, sap velocity was progressively reduced at all locations (Fig. 7). In the Lecciona catchment, although REW reduction influenced sap velocity, its effect on sap velocity varied between locations, likely resulting from the different soil moisture content between locations. In June, the high REW did not cause any differentiation in sap velocity response among locations, but in August, with reduced REW, sap velocity at the hilltop and midslope decreased, but this was not the case at the footslope. In September, despite the recharge of soil moisture by precipitation (Fig. 2f), sap velocity remained high only at the footslope and approached minimum values at the hilltop.
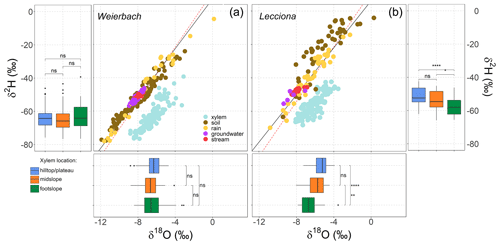
Figure 8Dual isotope plots showing soil water, rain, groundwater, stream, and xylem water isotopic composition sampled in the Weierbach catchment (a) and the Lecciona catchment (b). The boxplot shows the median (black line in the box), the interquartile range (extent of the box), the range (whiskers), and outliers (points) for xylem isotopic composition for the three sampling locations. The dashed and solid lines represent the global meteoric water line (GMWL) and the local meteoric water line (LMWL) for each site.
3.4 The isotopic composition of xylem, soil, groundwater, and precipitation
In the Weierbach catchment, xylem isotopic composition (157 samples; see Table A2) ranged between −8.8 and −3.1 ‰ for δ18O and −76.6 and −39.3 ‰ for δ2H. The mean isotopic composition was −6.4 ± 1.0 ‰ and −63.7 ± 7.1 ‰ in δ18O and δ2H, respectively. There was a marked temporal variability at all locations, with a typical higher isotopic composition during the sampling date from the dormant season (Fig. A4). We did not find any statistical difference in xylem isotopic composition between sampling locations (p = 0.2 for δ18O; p = 0.5, Kruskal–Wallis test) (Fig. 8). Soil water δ18O and δ2H showed the typical depth gradient, with heavier isotopic composition in the shallow layers (mean −5.5 ± 1.6 ‰ δ18O, −38.7 ± 9.6 ‰ δ2H) and lighter values in the deeper ones (−8.5 ± 1.4 ‰ δ18O, −55.7 ± 8.9 ‰ δ2H). The groundwater isotopic composition was stable over time and was statistically significantly lighter at the footslope (mean −8.5 ± 0.1 ‰ δ18O, −54.3 ± 1.1 ‰ δ2H) compared to at the midslope (mean −8.3 ± 0.3 ‰ δ18O, −56.9 ± 1.8 ‰ δ2H) and plateau (mean −8.5 ± 0.3 ‰ δ18O, −52.6 ± 2.3 ‰ δ2H) (p < 0.05, Kruskal–Wallis test).
In the Lecciona catchment, xylem water (96 samples; see Table A2) showed values between −8.0 and −3.5 ‰ for δ18O and −65.9 and −42.3 ‰ for δ2H. During the growing season, xylem water displayed a strong temporal pattern (Figs. A4 and A5). From May onwards, xylem water became heavier in both isotopes over time. After reaching the heaviest values on 11 June, it progressively became lighter (Figs. A4 and A5). The xylem water of trees located at the footslope (mean −6.5 ‰ δ18O and −57.6 ‰ δ2H) was significantly lighter in both isotopes (p < 0.01 δ18O, p < 0.02 δ2H – pairwise Wilcoxon test) compared to trees at the midslope (mean −5.9 ‰ δ18O and −53.9 ‰ δ2H) and hilltop (mean −5.4 ‰ δ18O and −51.6 ‰ δ2H) (Fig. 8). Soil water in Lecciona showed a marked depth gradient and no statistical difference among locations (data not shown). Soil isotopic composition was plotted above the LMWL, reflecting the heavy isotopic composition of the summer precipitation. Although we followed standard protocol for this analysis, and although the soil isotopic composition tracked the isotopic composition of precipitation, potential artefacts in the direct vapour equilibration method may have influenced these results. During the analyses, water vapour concentrations were above 28 000 ppm, and no methane was detected as an indication of potential microbial activity; both factors should ensure a reliable performance of the analysis (Gralher et al., 2021). However, we cannot exclude that shipping and storing procedures may have influenced the isotopic composition of soil samples. The isotopic composition of the groundwater sampled at the footslope displayed an average isotopic composition of −8.5 δ18O and −50.3 δ2H.
While topsoil (0–5 cm) isotopic composition displayed an average isotopic composition of −3.6 ± 1.9 ‰ for δ18O and −16.8 ± 13.9 ‰ for δ2H, the deepest soil depth sampled (40–60 cm) had an average isotopic composition of −6.5 ± 1.8 ‰ for δ18O and −31.9 ± 14.1 ‰ for δ2H. As in the Weierbach catchment, we did not find significant differences in soil water isotopic composition between sampling locations (p > 0.05, Wilcoxon rank sum test). At both sites, xylem water did not overlap with the potential water sources sampled (Fig. 8), but we observed a stronger deviation between xylem water and soil water in the Lecciona catchment (Fig. 8).
4.1 Drier conditions did not increase tree sensitivity to topography in the Weierbach catchment
According to our results, a lower availability of soil water in the Weierbach catchment in the 2020 growing season compared to in the 2019 growing season – which also marked the lowest recorded in the past 8 years – did not lead to a stronger sensitivity (i.e. stronger stomatal control and therefore reduced sap velocity) of tree water use to topography (Fig. A2). Instead, environmental controls (VPD, radiation, and REW) were the main predictors controlling sap velocity (Fig. A2). The 2020 growing season was characterized by lower precipitation and warmer conditions compared to 2019 (Table 2, Fig. 2), which is in line with the general drying and warming trend observed over the last decade in the catchment (Schoppach et al., 2021). Despite this, sap velocity in 2020 did not decrease compared to 2019, and sap velocities were even higher in May and September at all locations in 2020. Similarly, Gutierrez Lopez et al. (2021) found that, in Sweden, drier conditions did not affect transpiration compared to a wetter previous year because small precipitation events were sufficient enough to recharge soil moisture. The gradual decrease in soil moisture content concurrently with an increase in VPD caused a reduction in normalized sap velocity, especially at the footslope location in both years (Fig. 6 and 7). We found that maximum sap velocities were reached for the same VPD range (1.0–1.5 kPa). However, the number of days with VPD higher than 1.5 kPa doubled in 2020, which, coupled with the depletion in soil water, might be the cause for the early leaf yellowing and defoliation observed from mid-August at our site. According to Walthert et al. (2021), early defoliation in beech can be used as a predictor for crown die-back in the following year. In fact, the effects of water shortage cannot be confined to the study year alone because the carry-over effects from current conditions will be expressed over the next seasons (Walthert et al., 2021).
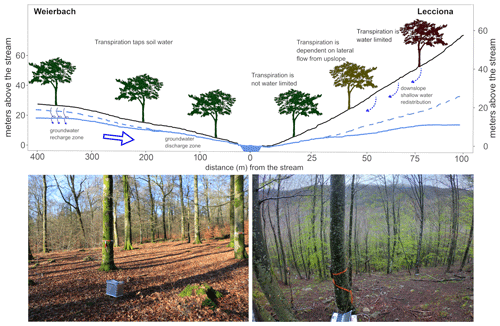
Figure 9Conceptual graph showing the individual monitoring locations at each catchment and the ecohydrological processes taking place. Photos of the hillslopes are also shown for each study site from the plateau and hilltop positions. The terrain profiles were extracted from the digital terrain model (DEM – grid size 5 and 10 m in the Weierbach catchment and the Lecciona catchment, respectively). In the Weierbach catchment, the homogeneous edaphic conditions lead to a lack of statistical difference in transpiration across the landscape. On the contrary, in the Lecciona catchment, the subsurface structure combined with the steep slope creates a gradient of water stress (represented as a colour gradient in the trees).
Consistently with previous observations at the study site for 2019 (Fabiani et al., 2022b), the high vertical hydraulic conductivity in the Weierbach catchment (Glaser et al., 2016; Gourdol et al., 2021) does not promote a shallow lateral subsurface from the plateau to the footslope (Fig. 9). Instead, deeper hydrological flow paths dominate (Rodriguez and Klaus, 2019), leading to a shallow and rather stable groundwater table at the footslope (Fabiani et al., 2022b). However, groundwater proximity to the soil surface did not have any influence on tree physiological response even during the dry conditions in 2020 (Fig. 2g). The 2019 minimum groundwater table depths at the plateau and midslope locations fell in 2020, reaching a level below the typical rooting depth of F. sylvatica (0.83 ± 0.46) (Fan et al., 2017). This evidence, combined with the lack of statistical difference in xylem isotopic composition between locations (Fig. 8), supports the conclusion that there is a negligible role of groundwater as a water source for beech trees in the Weierbach catchment. Similarly, at a different study site, Weemstra et al. (2013) did not observe any impact of interannual variation in groundwater depth on beech growth rates. Although plants can grow roots deeper in the soil profile as a response to water limitation (Bréda et al., 2006), beech trees show little plasticity and rely on shallow soil storage regardless of the drought conditions (Gessler et al., 2022; Volkmann et al., 2016). This result emphasizes the importance of occasional rainfall to wet the topsoil, where most of the fine roots of F. sylvatica are located (Coners and Leuschner, 2005; Meier and Leuschner, 2008).
Another potential water source to buffer water shortage is stem water (Tyree and Yang, 1990). The progressive dehydration of the xylem from winter to summer suggests that the stem internal water storage may have acted as an additional water source for tree transpiration (Figs. 4 and A3). Seasonal changes in stem water content resulting from the use of storage water have already been observed in other studies, with progressive water depletion over summer and refilling after leaf fall (Tyree and Zimmermann, 2002; Fabiani et al., 2022a). The relevance of stem-stored water has already been highlighted in other studies which showed that the internal water plays a crucial role in the water balance of beech trees, improving their ability to cope with water stresses (Betsch et al., 2011; Köcher et al., 2013; Matheny et al., 2017). While we observed significantly lower wood moisture on the plateau compared to other locations in the Weierbach catchment (and not in the Lecciona) (Figs. 4 and A3), we acknowledge that our measurements provided only snapshots of xylem water status and cannot capture fine-scale differences that could exist across the landscape.
4.2 Topography influences the duration of the growing season in the Lecciona catchment
In contrast to the Weierbach catchment, the topography in the Lecciona catchment had a significant effect on sap velocity but only towards the end of the growing season when the system was dry (Fig. A2). We observed that sap velocity remained significantly higher at the footslope compared to at other locations during this time. This evidence supports our hypothesis that the combination of steep topography and subsurface characteristics that promote fast and shallow subsurface flow at the Lecciona study site (Mary et al., 2023) enhances the variability in energy and water inputs (Fig. 9). The higher soil moisture content at the bottom of the slope compared to at the upslope locations in the Lecciona catchment (Fig. 2f) leads to spatially different physiological responses in trees. Additionally, the thick litter accumulated downslope from the upper slope (Fig. 9) might have acted as a barrier, reducing soil evaporation at the footslope. In the Lecciona catchment, until July, the relationship between sap velocity and VPD was similar among hillslope positions (Figs. 7 and 6), suggesting that soil water supply was sufficient to compensate for evapotranspiration losses (Bréda and Granier, 1996). However, in August, the reduced soil water availability at the hilltop and midslope resulted in a down-regulation of sap velocity (Figs. 6 and 7). When more favourable conditions were restored as a result of rainfall events in September that recharged the upper soil (Fig. 2f), sap velocities at the hilltop and midslope locations did not recover the pre-drought transpiration (Figs. 6 and 7). At this time, hillslope topography had a significant positive effect on sap velocity, leading to sustained transpiration at the footslope (Fig. A2). This result supports the anticipated effects of landscape characteristics on tree transpiration, leading to more conservative hydraulic behaviour in upper-topographic positions due to the xeric conditions at the Lecciona catchment (Kumagai et al., 2008; Song et al., 2022). We also observed that the decrease in soil water content at the upslope location led to a progressively shorter vegetative-season moving for upper slope positions at the Lecciona footslope (Fig. 5). This result might also be interpreted as evidence for the physiological acclimation of beech trees located at the hilltop and midslope locations. The drier and more evaporatively demanding conditions given by the higher solar radiation at the midslope and hilltop areas compared to at the footslope (Méndez-Toribio et al., 2017) could cause trees in higher topographic positions to experience shorter growing seasons in order to withstand decreasing water supply (Fig. 5). Similarly, Méndez-Toribio et al. (2017) observed a shorter growth time window in the upper part of slopes as a strategy to mitigate drought via leaf shedding or by the use of internal water storage. The statistical difference in xylem isotopic composition between locations observed in the Lecciona catchment but not in the Weierbach catchment (Fig. 8) might suggest that trees capitalize on different water sources with contrasting isotopic compositions to adapt to spatial changes in water availability. We found significantly heavier xylem isotopic composition at the hilltop and midslope locations compared to the at footslope location in the Lecciona catchment (Figs. A4 and A5), but we found no differences in the soil isotopic composition, suggesting that the difference in xylem isotopes is not related to spatial differences in soil isotopes of the upper 60 cm of the soil. This might indicate a greater use of shallow water sources in the upslope locations due to the inaccessibility of the deep aquifer. However, from the soil isotopic composition (Fig. 8), we cannot confirm this interpretation due to the large offset between xylem and soil composition and groundwater and stream water isotopic composition, as has been commonly observed in other studies (Barbeta et al., 2019; Poca et al., 2019; de la Casa et al., 2022; Schreel et al., 2023). This topic has received increasing attention in the recent literature, but no ubiquitous explanations have been confirmed. In the Lecciona catchment, we suggest that footslope trees, compared to those at upslope locations, benefit from the greater accessibility and availability of water stored in the deeper soil layers and in the weathered bedrock, which generally displays lighter isotopic composition compared to shallow soil water.
We observed that transpiration responses to VPD differed between the two study sites despite a similar soil moisture range. This is because different soil textures lead to different water availabilities for plant water use. The loamy soil texture in the Weierbach catchment translates into more negative soil water potential compared to the sandy loam in the Lecciona catchment for similar soil moisture content values. For this reason, since soil moisture retention curves are missing at our study sites, we rely on REW to compare the Lecciona and Weierbach catchments. While maximum sap velocities were markedly reduced for VPD above 1.5 kPa in the Weierbach catchment (Fig. 7), in the Lecciona catchment, sap velocity at the hilltop remained high, even for VPD values above 2 kPa (Fig. 3). This suggests that trees at the footslope location in the Lecciona catchment were not water-limited and likely had access to water stored in deep soil or saprolite. When compared to other species, F. sylvatica is generally considered to display an isohydric behaviour (Fabiani et al., 2022b; Gessler, 2021; Magh et al., 2020; Martínez-Vilalta and Garcia-Forner, 2017), which means that, with decreasing VPD, beech trees tend to close their stomata to minimize water loss (Grossiord et al., 2017b). However, the different sap velocity responses to VPD observed at our sites (Figs. 6 and 7) suggest that the hydraulic behaviour of this species could also change from site to site as an adaptation to hydrometeorological conditions and landscape features. Indeed, water use strategies within the same species can vary both spatially and temporally according to the coupling between soil moisture, atmospheric drivers, and morphological adjustments (Kannenberg et al., 2022). Additionally, as already pointed out by Hochberg et al. (2018), when comparing data from multiple sites, we do not always have the capability to separate environmental and genotypic variability. The wide distribution range occupied by F. sylvatica in Europe results from the high plasticity of this species and the phenotypic variations, including those relating to drought resistance (Stojnić et al., 2018). Previous literature has already emphasized that trees' hydraulic strategies should not strictly be considered to be a dichotomy between iso- and anisohydric behaviour but rather a continuum between the two (Martínez-Vilalta et al., 2014). Despite some evidence regarding the adaptation potential of beech trees to changing environmental conditions, speculated upon in a study that revealed the high plasticity capacity of the stem hydraulic system in F. sylvatica over decades (Zimmermann et al., 2021), it remains unclear how a long-term increase in soil and air dryness may impact this species, which is vulnerable to drought (Walthert et al., 2021), across its wide distribution range.
So far, no study has explored the influence of topography on tree water use within the same species growing in diverse climatic settings. In the present study, we found that surface topography and hillslope structure result in varying sap velocity responses to environmental controls (VPD and REW) but not consistently across landscapes (Fig. 9), as initially hypothesized. In the Weierbach catchment, the homogeneous growing conditions across the slope and the vertical connectivity of the subsurface structure result in uniform physiological responses to environmental controls among trees, even during the drier conditions of 2020 compared to 2019. Contrarily, in the Lecciona catchment, we observed a stronger edaphic and environmental gradient across the hillslope topography, where upslope locations become water-limited by the interplay of multiple factors. In the Lecciona catchment, water redistribution downslope through shallow subsurface flow results in a longer growing season for trees located at the footslope, where growing conditions are more favourable. These contrasting results in the two investigated slopes suggest that, in landscapes where the hydraulic and climatic gradients are stronger, the physiological response of the same forest species among locations may be more spatially variable. Contrarily to what was initially hypothesized, the physiological activity of beech trees was not constrained when compared to the central European population in the Weierbach catchment, likely due to the plasticity of this species. Therefore, the present work contributes to emphasizing that different environmental conditions drive different physiological responses in space and time within the same species. Future work carrying out a cross-site comparison may take into account the effect of genotypic variability, which may lead to differing physiological responses for similar environmental conditions. Having information on the drought resistance traits across a species distribution range will be crucial to assess species responses in the face of the current climate change.
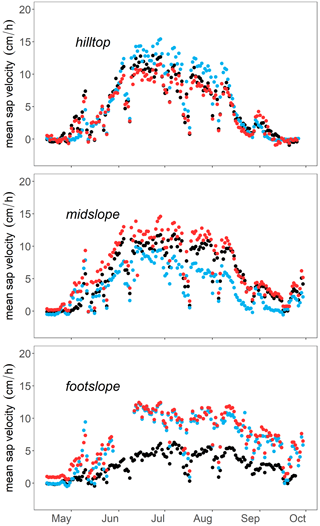
Figure A1Sap velocity (cm h−1) of beech at three sampling areas (hilltop, midslope, footslope) in the Lecciona catchment; each colour corresponds to one equipped tree. Missing data are the result of power interruption. When possible, data gaps were filled by interpolation. The shaded grey area represents the standard deviation from the mean soil moisture across three monitoring locations in the Lecciona catchment.
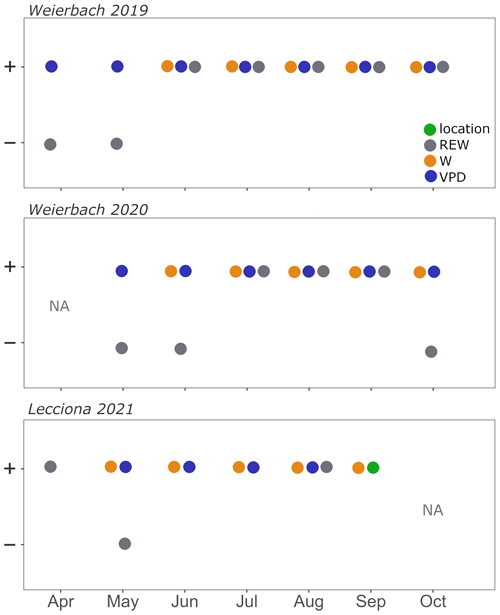
Figure A2Summary of significant predictors explaining the variability in sap velocity for the Weierbach catchment and the Lecciona catchment. Significance of the predictors has been evaluated with linear-mixing model analysis. Fixed effects used in the model include topographic location (hilltop or plateau, midslope, footslope), relative extractable water (REW), radiation (W m−2), and vapour pressure deficit (VPD). The + and − signs correspond to a significant increase or decrease, respectively, in sap velocity following the increase in the predictor.
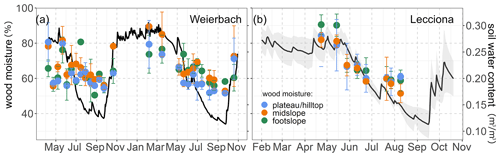
Figure A3Temporal pattern of wood moisture content (%) and volumetric soil moisture content in the Weierbach catchment (a) and in the Lecciona catchment (b). Solid dots represent the mean, and the whiskers indicate the confidence interval (95 %) of wood moisture measured at the three sampling locations. In the Weierbach catchment, we used the average soil moisture content over the four depths, while in the Lecciona catchment, we used the average soil moisture over the three monitored locations. The shaded grey area represents the standard deviation from the mean soil moisture across three monitoring locations in the Lecciona catchment.
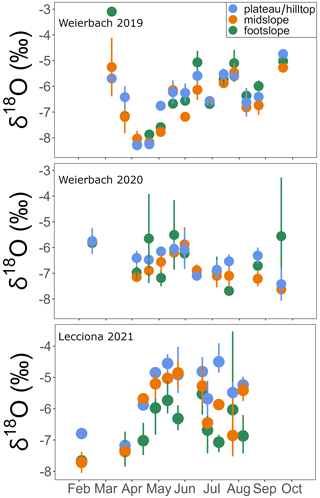
Figure A4δ18O of xylem water for each sampling campaign across the 2019 and 2020 growing seasons in the Weierbach catchment and over the 2021 growing season in the Lecciona catchment. Data were averaged by location. Solid dots represent the mean, and the whiskers indicate the confidence interval (95 %).
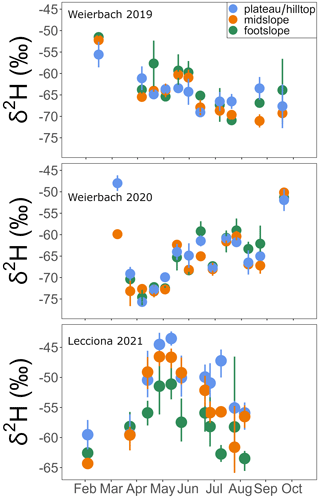
Figure A5δ2H of xylem water for each sampling campaign across the 2019 and 2020 growing seasons in the Weierbach catchment and over the 2021 growing season in the Lecciona catchment. Data were averaged by location. Solid dots represent the mean and the whiskers indicate the confidence interval (95 %).
The data that support the findings of this study are available for the Lecciona catchment (https://doi.org/10.5281/ZENODO.8328006, Fabiani et al., 2023b) and the Weierbach catchment (https://doi.org/10.5281/ZENODO.8326112, Fabiani et al., 2023a) in Zenodo repositories.
GF: conceptualization, funding acquisition, investigation, formal analysis, visualization, and writing – original draft. JK: conceptualization, funding acquisition, supervision, validation, writing – review and editing, and project administration. DP: conceptualization, funding acquisition, supervision, and writing – review and editing.
The contact author has declared that none of the authors has any competing interests.
Publisher's note: Copernicus Publications remains neutral with regard to jurisdictional claims made in the text, published maps, institutional affiliations, or any other geographical representation in this paper. While Copernicus Publications makes every effort to include appropriate place names, the final responsibility lies with the authors.
This work was supported by the Luxembourg National Research Fund (grant no. AFR/STEP-UP ID 12546983), the Italian Ministry for University and Research (WATZON grant no. 2017SL7ABC, WATERSTEM grant no. 20202WF53Z), and the National Research Council – CNR (Carbon and water cycles interactions during drought and their impact on WAter and ForEst Resources in the Mediterranean region – WAFER). We thank Enrico Bonanno, Adnan Moussa, Remy Schoppach, Jean Francois Iffly, Laurent Gourdol, Cyrille Tailliez, Matteo Verdone, Francesca Sofia Manca di Villahermosa, Andrea Dani, and Ilaria Zorzi for their help during the field work and for the fruitful discussions. We express our sincere gratitude to Richard Keim, Ilja van Meerveld, Claudia Cocozza, Adrià Barbeta, and Laurent Pfister for their invaluable insights regarding this paper, which enhanced the quality of this work. We thank Conrad Jackisch and the anonymous reviewer for their valuable feedback during the revision of this work.
This research has been supported by the Fonds National de la Recherche Luxembourg (grant no. AFR/STEP-UP ID 12546983).
This paper was edited by Ralf Loritz and reviewed by Conrad Jackisch and one anonymous referee.
Allen, C. D., Macalady, A. K., Chenchouni, H., Bachelet, D., McDowell, N., Vennetier, M., Kitzberger, T., Rigling, A., Breshears, D. D., Hogg, E. T., Gonzalez, P., Fensham, R., Zhang, Z., Castro, J., Demidova, N., Lim, J.-H., Allard, G., Running, S. W., Semerci, A., and Cobb, N.: A global overview of drought and heat-induced tree mortality reveals emerging climate change risks for forests, Forest Ecol. Manag., 259, 660–684, https://doi.org/10.1016/j.foreco.2009.09.001, 2010.
Barbeta, A., Jones, S. P., Clavé, L., Wingate, L., Gimeno, T. E., Fréjaville, B., Wohl, S., and Ogée, J.: Unexplained hydrogen isotope offsets complicate the identification and quantification of tree water sources in a riparian forest, Hydrol. Earth Syst. Sci., 23, 2129–2146, https://doi.org/10.5194/hess-23-2129-2019, 2019.
Barrett, D. J., Hatton, T. J., Ash, J. E., and Ball, M. C.: Evaluation of the heat pulse velocity technique for measurement of sap flow in rainforest and eucalypt forest species of south-eastern Australia, Plant Cell Environ., 18, 463–469, https://doi.org/10.1111/j.1365-3040.1995.tb00381.x, 1995.
Bates, D., Mächler, M., Bolker, B., and Walker, S.: Fitting Linear Mixed-Effects Models Using lme4, J. Stat. Softw., 67, 1–48, https://doi.org/10.18637/jss.v067.i01, 2015.
Betsch, P., Bonal, D., Breda, N., Montpied, P., Peiffer, M., Tuzet, A., and Granier, A.: Drought effects on water relations in beech: The contribution of exchangeable water reservoirs, Agr. Forest Meteorol., 151, 531–543, https://doi.org/10.1016/j.agrformet.2010.12.008, 2011.
Bosch, D. D., Marshall, L. K., and Teskey, R.: Forest transpiration from sap flux density measurements in a Southeastern Coastal Plain riparian buffer system, Agr. Forest Meteorol., 187, 72–82, https://doi.org/10.1016/j.agrformet.2013.12.002, 2014.
Bréda, N. and Granier, A.: Intra- and interannual variations of transpiration, leaf area index and radial growth of a sessile oak stand (Quercus petraea), Ann. Sci. Forest., 53, 521–536, https://doi.org/10.1051/forest:19960232, 1996.
Bréda, N., Huc, R., Granier, A., and Dreyer, E.: Temperate forest trees and stands under severe drought: a review of ecophysiological responses, adaptation processes and long-term consequences, Ann. For. Sci., 63, 625–644, https://doi.org/10.1051/forest:2006042, 2006.
Burgess, S. S. O., Adams, M. A., Turner, N. C., Beverly, C. R., Ong, C. K., Khan, A. A. H., and Bleby, T. M.: An improved heat pulse method to measure low and reverse rates of sap flow in woody plants, Tree Physiol., 21, 589–598, 2001.
Cavin, L. and Jump, A. S.: Highest drought sensitivity and lowest resistance to growth suppression are found in the range core of the tree Fagus sylvatica L. not the equatorial range edge, Glob. Change Biol., 23, 362–379, https://doi.org/10.1111/gcb.13366, 2017.
Choat, B., Jansen, S., Brodribb, T. J., Cochard, H., Delzon, S., Bhaskar, R., Bucci, S. J., Feild, T. S., Gleason, S. M., Hacke, U. G., Jacobsen, A. L., Lens, F., Maherali, H., Martínez-Vilalta, J., Mayr, S., Mencuccini, M., Mitchell, P. J., Nardini, A., Pittermann, J., Pratt, R. B., Sperry, J. S., Westoby, M., Wright, I. J., and Zanne, A. E.: Global convergence in the vulnerability of forests to drought, Nature, 491, 752–755, https://doi.org/10.1038/nature11688, 2012.
Coners, H. and Leuschner, C.: In situ measurement of fine root water absorption in three temperate tree species – Temporal variability and control by soil and atmospheric factors, Basic Appl. Ecol., 6, 395–405, https://doi.org/10.1016/j.baae.2004.12.003, 2005.
de la Casa, J., Barbeta, A., Rodríguez-Uña, A., Wingate, L., Ogée, J., and Gimeno, T. E.: Isotopic offsets between bulk plant water and its sources are larger in cool and wet environments, Hydrol. Earth Syst. Sci., 26, 4125–4146, https://doi.org/10.5194/hess-26-4125-2022, 2022.
Dobrowski, S. Z.: A climatic basis for microrefugia: The influence of terrain on climate, Glob. Change Biol., 17, 1022–1035, https://doi.org/10.1111/j.1365-2486.2010.02263.x, 2011.
Elliott, K. J., Miniat, C. F., Pederson, N., and Laseter, S. H.: Forest tree growth response to hydroclimate variability in the southern Appalachians, Glob. Change Biol., 21, 4627–4641, https://doi.org/10.1111/gcb.13045, 2015.
Fabiani, G., Penna, D., Barbeta, A., and Klaus, J.: Sapwood and heartwood are not isolated compartments: Consequences for isotope ecohydrology, Ecohydrology, 15, e2478, https://doi.org/10.1002/eco.2478, 2022a.
Fabiani, G., Schoppach, R., Penna, D., and Klaus, J.: Transpiration patterns and water use strategies of beech and oak trees along a hillslope, Ecohydrology, 15, 1–18, https://doi.org/10.1002/eco.2382, 2022b.
Fabiani, G., Schoppach, R., Moussa, A., Pfister, L., Penna, D., and Klaus, J.: STEP UP project: the Weierbach dataset 2019–2020, Zenodo [data set], https://doi.org/10.5281/ZENODO.8326112, 2023a.
Fabiani, G., Verdone, M., Manca di Villahermosa, F. S., Pfister, L., Klaus, J., and Penna, D.: STEP UP project: the Lecciona dataset 2021, Zenodo [data set], https://doi.org/10.5281/ZENODO.8328006, 2023b.
Fan, Y., Miguez-Macho, G., Jobbágy, E. G., Jackson, R. B., and Otero-Casal, C.: Hydrologic regulation of plant rooting depth, P. Natl. Acad. Sci. USA, 114, 10572–10577, https://doi.org/10.1073/pnas.1712381114, 2017.
Gessler, A.: Water transport in trees—the importance of radial and circumferential transport, Tree Physiol., 1–3, https://doi.org/10.1093/treephys/tpab131, 2021.
Gessler, A., Bächli, L., Rouholahnejad Freund, E., Treydte, K., Schaub, M., Haeni, M., Weiler, M., Seeger, S., Marshall, J., Hug, C., Zweifel, R., Hagedorn, F., Rigling, A., Saurer, M., and Meusburger, K.: Drought reduces water uptake in beech from the drying topsoil, but no compensatory uptake occurs from deeper soil layers, New Phytol., 233, 194–206, https://doi.org/10.1111/nph.17767, 2022.
Gimenez, B. O., Jardine, K. J., Higuchi, N., Negrón-Juárez, R. I., Sampaio-Filho, I. d. J., Cobello, L. O., Fontes, C. G., Dawson, T. E., Varadharajan, C., Christianson, D. S., Spanner, G. C., Araújo, A. C., Warren, J. M., Newman, B. D., Holm, J. A., Koven, C. D., McDowell, N. G., and Chambers, J. Q.: Species-specific shifts in diurnal sap velocity dynamics and hysteretic behavior of ecophysiological variables during the 2015–2016 El Niño event in the amazon forest, Front. Plant Sci., 10, 1–16, https://doi.org/10.3389/fpls.2019.00830, 2019.
Giménez, C., Gallardo, M., and Thompson, R.: Plant–Water Relations, in: Reference Module in Earth Systems and Environmental Sciences, Elsevier, https://doi.org/10.1016/B978-0-12-409548-9.05257-X, pp. 1–8, May, 2013.
Glaser, B., Klaus, J., Frei, S., Frentress, J., Pfister, L., and Hopp, L.: On the value of surface saturated area dynamics mapped with thermal infrared imagery for modeling the hillslope–riparian–stream continuum, Water Resour. Res., 52, 8317–8342, https://doi.org/10.1002/2015WR018414, 2016.
Gourdol, L., Clément, R., Juilleret, J., Pfister, L., and Hissler, C.: Exploring the regolith with electrical resistivity tomography in large-scale surveys: electrode spacing-related issues and possibility, Hydrol. Earth Syst. Sci., 25, 1785–1812, https://doi.org/10.5194/hess-25-1785-2021, 2021.
Gralher, B., Herbstritt, B., and Weiler, M.: Technical note: Unresolved aspects of the direct vapor equilibration method for stable isotope analysis (δ18O, δ2H) of matrix-bound water: unifying protocols through empirical and mathematical scrutiny, Hydrol. Earth Syst. Sci., 25, 5219–5235, https://doi.org/10.5194/hess-25-5219-2021, 2021.
Granier, A., Bréda, N., Biron, P., and Villette, S.: A lumped water balance model to evaluate duration and intensity of drought constraints in forest stands, Ecol. Model., 116, 269–283, https://doi.org/10.1016/S0304-3800(98)00205-1, 1999.
Granier, A., Reichstein, M., Bréda, N., Janssens, I. A., Falge, E., Ciais, P., Grünwald, T., Aubinet, M., Berbigier, P., Bernhofer, C., Buchmann, N., Facini, O., Grassi, G., Heinesch, B., Ilvesniemi, H., Keronen, P., Knohl, A., Köstner, B., Lagergren, F., Lindroth, A., Longdoz, B., Loustau, D., Mateus, J., Montagnani, L., Nys, C., Moors, E., Papale, D., Peiffer, M., Pilegaard, K., Pita, G., Pumpanen, J., Rambal, S., Rebmann, C., Rodrigues, A., Seufert, G., Tenhunen, J., Vesala, T., and Wang, Q.: Evidence for soil water control on carbon and water dynamics in European forests during the extremely dry year: 2003, Agr. Forest Meteorol., 143, 123–145, https://doi.org/10.1016/j.agrformet.2006.12.004, 2007.
Green, S., Clothier, B., and Jardine, B.: Theory and Practical Application of Heat Pulse to Measure Sap Flow, Agron. J., 95, 1371–1379, https://doi.org/10.2134/agronj2003.1371, 2003.
Grossiord, C., Sevanto, S., Borrego, I., Chan, A. M., Collins, A. D., Dickman, L. T., Hudson, P. J., McBranch, N., Michaletz, S. T., Pockman, W. T., Ryan, M., Vilagrosa, A., and McDowell, N. G.: Tree water dynamics in a drying and warming world, Plant Cell Environ., 40, 1861–1873, https://doi.org/10.1111/pce.12991, 2017a.
Grossiord, C., Sevanto, S., Dawson, T. E., Adams, H. D., Collins, A. D., Dickman, L. T., Newman, B. D., Stockton, E. A., and McDowell, N. G.: Warming combined with more extreme precipitation regimes modifies the water sources used by trees, New Phytol., 213, 584–596, https://doi.org/10.1111/nph.14192, 2017b.
Gutierrez Lopez, J., Tor-ngern, P., Oren, R., Kozii, N., Laudon, H., and Hasselquist, N. J.: How tree species, tree size, and topographical location influenced tree transpiration in northern boreal forests during the historic 2018 drought, Glob. Change Biol., 27, 3066–3078, https://doi.org/10.1111/gcb.15601, 2021.
Hahm, W. J., Dietrich, W. E., and Dawson, T. E.: Controls on the distribution and resilience of Quercus garryana: ecophysiological evidence of oak's water-limitation tolerance, Ecosphere, 9, e02218, https://doi.org/10.1002/ecs2.2218, 2018.
Hawthorne, S. and Miniat, C. F.: Topography may mitigate drought effects on vegetation along a hillslope gradient, Ecohydrology, 11, e1825, https://doi.org/10.1002/eco.1825, 2018.
Hissler, C., Martínez-Carreras, N., Barnich, F., Gourdol, L., Iffly, J. F., Juilleret, J., Klaus, J., and Pfister, L.: The Weierbach experimental catchment in Luxembourg: A decade of critical zone monitoring in a temperate forest – from hydrological investigations to ecohydrological perspectives, Hydrol. Process., 35, 1–7, https://doi.org/10.1002/hyp.14140, 2021.
Hochberg, U., Rockwell, F. E., Holbrook, N. M., and Cochard, H.: Iso/Anisohydry: A Plant–Environment Interaction Rather Than a Simple Hydraulic Trait, Trends Plant Sci., 23, 112–120, https://doi.org/10.1016/j.tplants.2017.11.002, 2018.
Hogg, E. H., Brandt, J. P., and Michaelian, M.: Impacts of a regional drought on the productivity, dieback, and biomass of western Canadian aspen forests, Can. J. Forest Res., 38, 1373–1384, https://doi.org/10.1139/X08-001, 2008.
Houston Durrant, T., de Rigo, D., and Caudullo, G.: Fagus sylvatica and other beeches in Europe: distribution, habitat, usage and threats, in: European Atlas of Forest Tree Species, edited by: San-Miguel-Ayanz, J., de Rigo, D., Caudullo, G., Houston Durrant, T., and Mauri, A., Publ. Off. EU, Luxembourg, ISBN 978-92-76-17290-1, 2016.
Hoylman, Z. H., Jencso, K. G., Hu, J., Martin, J. T., Holden, Z. A., Seielstad, C. A., and Rowell, E. M.: Hillslope Topography Mediates Spatial Patterns of Ecosystem Sensitivity to Climate, J. Geophys. Res.-Biogeo., 123, 353–371, https://doi.org/10.1002/2017JG004108, 2018.
Jencso, K. G., McGlynn, B. L., Gooseff, M. N., Wondzell, S. M., Bencala, K. E., and Marshall, L. A.: Hydrologic connectivity between landscapes and streams: Transferring reach- and plot-scale understanding to the catchment scale, Water Resour. Res., 45, 1–16, https://doi.org/10.1029/2008WR007225, 2009.
Kannenberg, S. A., Guo, J. S., Novick, K. A., Anderegg, W. R., Feng, X., Kennedy, D., Konings, A. G., Martínez-Vilalta, J., and Matheny, A. M.: Opportunities, challenges and pitfalls in characterizing plant water-use strategies, Funct. Ecol., 36, 24–37, https://doi.org/10.1111/1365-2435.13945, 2022.
Klaus, J. and Jackson, C. R.: Interflow Is Not Binary: A Continuous Shallow Perched Layer Does Not Imply Continuous Connectivity, Water Resour. Res., 54, 5921–5932, https://doi.org/10.1029/2018WR022920, 2018.
Klaus, J., Chun, K. P., and Stumpp, C.: Temporal trends in δ18O composition of precipitation in Germany: Insights from time series modelling and trend analysis, Hydrol. Process., 29, 2668–2680, https://doi.org/10.1002/hyp.10395, 2015.
Klaus, J., Monk, W. A., Zhang, L., and Hannah, D. M.: Ecohydrological interactions during drought, Ecohydrology, 15, 1–7, https://doi.org/10.1002/eco.2456, 2022.
Köcher, P., Horna, V., and Leuschner, C.: Stem water storage in five coexisting temperate broad-leaved tree species: Significance, temporal dynamics and dependence on tree functional traits, Tree Physiol., 33, 817–832, https://doi.org/10.1093/treephys/tpt055, 2013.
Kreuzwieser, J. and Rennenberg, H.: Molecular and physiological responses of trees to waterlogging stress, Plant Cell Environ., 37, 2245–2259, https://doi.org/10.1111/pce.12310, 2014.
Kumagai, T., Tateishi, M., Shimizu, T., and Otsuki, K.: Transpiration and canopy conductance at two slope positions in a Japanese cedar forest watershed, Agr. Forest Meteorol., 148, 1444–1455, https://doi.org/10.1016/j.agrformet.2008.04.010, 2008.
Li, K. and Knighton, J.: Characterizing the heterogeneity of eastern hemlock xylem water isotopic compositions: Implications for the design of plant water uptake studies, Ecohydrology, 16, e2571, https://doi.org/10.1002/eco.2571, 2023.
Magh, R.-K., Eiferle, C., Burzlaff, T., and Dannenmann, M.: Competition for water rather than facilitation in mixed beech-fir forests after drying-wetting cycle, J. Hydrol., 587, 124944, https://doi.org/10.1016/j.jhydrol.2020.124944, 2020.
Martínez-Vilalta, J. and Garcia-Forner, N.: Water potential regulation, stomatal behaviour and hydraulic transport under drought: deconstructing the iso/anisohydric concept, Plant Cell Environ., 40, 962–976, https://doi.org/10.1111/pce.12846, 2017.
Martínez-Vilalta, J., Poyatos, R., Aguadé, D., Retana, J., and Mencuccini, M.: A new look at water transport regulation in plants, New Phytol., 204, 105–115, https://doi.org/10.1111/nph.12912, 2014.
Mary, B., Kaffas, K., Censini, M., Manca di Villahermosa, F. S., Dani, A., Verdone, M., Preti, F., Trucchi, P., Penna, D., and Cassiani, G.: Supporting subsurface preferential flow in a small forested catchment from geophysical data and hydrological modelling, EGU General Assembly 2023, Vienna, Austria, 24–28 April 2023, EGU23-5954, https://doi.org/10.5194/egusphere-egu23-5954, 2023.
Matheny, A. M., Garrity, S. R., and Bohrer, G.: The calibration and use of capacitance sensors to monitor stem water content in trees, JOVE-J. Vis. Exp., 2017, 1–10, https://doi.org/10.3791/57062, 2017.
McDowell, N., Pockman, W. T., Allen, C. D., Breshears, D. D., Cobb, N., Kolb, T., Plaut, J., Sperry, J., West, A., Williams, D. G., and Yepez, E. A.: Mechanisms of plant survival and mortality during drought: why do some plants survive while others succumb to drought?, New Phytol., 178, 719–739, https://doi.org/10.1111/j.1469-8137.2008.02436.x, 2008.
Meier, I. C. and Leuschner, C.: Belowground drought response of European beech: Fine root biomass and carbon partitioning in 14 mature stands across a precipitation gradient, Glob. Change Biol., 14, 2081–2095, https://doi.org/10.1111/j.1365-2486.2008.01634.x, 2008.
Méndez-Toribio, M., Ibarra-Manríquez, G., Navarrete-Segueda, A., and Paz, H.: Topographic position, but not slope aspect, drives the dominance of functional strategies of tropical dry forest trees, Environ. Res. Lett., 12, 085002, https://doi.org/10.1088/1748-9326/aa717b, 2017.
Meusburger, K., Trotsiuk, V., Schmidt-Walter, P., Baltensweiler, A., Brun, P., Bernhard, F., Gharun, M., Habel, R., Hagedorn, F., Köchli, R., Psomas, A., Puhlmann, H., Thimonier, A., Waldner, P., Zimmermann, S., and Walthert, L.: Soil-plant interactions modulated water availability of Swiss forests during the 2015 and 2018 droughts, Glob. Change Biol., pp. 0–3, https://doi.org/10.1111/gcb.16332, 2022. Murphy, P. C., Knowles, J. F., Moore, D. J., Anchukaitis, K., Potts, D. L., and Barron-Gafford, G. A.: Topography influences species-specific patterns of seasonal primary productivity in a semiarid montane forest, Tree Physiol., 40, 1343–1354, https://doi.org/10.1093/TREEPHYS/TPAA083, 2020.
Oberhuber, W. and Kofler, W.: Topographic influences on radial growth of Scots pine (Pinus sylvestris L.) at small spatial scales, Plant Ecol., 146, 231–240, 2000.
Obladen, N., Dechering, P., Skiadaresis, G., Tegel, W., Keßler, J., Höllerl, S., Kaps, S., Hertel, M., Dulamsuren, C., Seifert, T., Hirsch, M., and Seim, A.: Tree mortality of European beech and Norway spruce induced by 2018–2019 hot droughts in central Germany, Agr. Forest Meteorol., 307, 108 482, https://doi.org/10.1016/j.agrformet.2021.108482, 2021.
Orlowski, N., Breuer, L., and Mcdonnell, J. J.: Critical issues with cryogenic extraction of soil water for stable isotope analysis, Ecohydrology, 9, 3–10, https://doi.org/10.1002/eco.1722, 2016.
Peck, E. C.: The sap of moisture in wood, US Dept. Agr. For. Serv. For. Prod. Lab., 1953.
Penna, D., Borga, M., Norbiato, D., and Dalla Fontana, G.: Hillslope scale soil moisture variability in a steep alpine terrain, J. Hydrol., 364, 311–327, https://doi.org/10.1016/j.jhydrol.2008.11.009, 2009.
Penna, D., Stenni, B., Šanda, M., Wrede, S., Bogaard, T. A., Michelini, M., Fischer, B. M. C., Gobbi, A., Mantese, N., Zuecco, G., Borga, M., Bonazza, M., Sobotková, M., Čejková, B., and Wassenaar, L. I.: Technical Note: Evaluation of between-sample memory effects in the analysis of δ2H and δ18O of water samples measured by laser spectroscopes, Hydrol. Earth Syst. Sci., 16, 3925–3933, https://doi.org/10.5194/hess-16-3925-2012, 2012.
Penna, D., Mantese, N., Hopp, L., Dalla Fontana, G., and Borga, M.: Spatio-temporal variability of piezometric response on two steep alpine hillslopes, Hydrol. Process., 29, 198–211, https://doi.org/10.1002/hyp.10140, 2015.
Pfister, L., Bonanno, E., Fabiani, G., Gourdol, L., Hissler, C., Huck, V., Iffly, J. F., Keim, R., Martínez-Carreras, N., Mestdagh, X., Montemagno, A., Penna, D., Schymanski, S., and Zehe, E.: Fast motion view of a headwater creek—A hydrological year seen through time-lapse photography, Hydrol. Process., 37, 1–10, https://doi.org/10.1002/hyp.15026, 2023.
Poca, M., Coomans, O., Urcelay, C., Zeballos, S. R., Bodé, S., and Boeckx, P.: Isotope fractionation during root water uptake by Acacia caven is enhanced by arbuscular mycorrhizas, Plant Soil, 441, 485–497, https://doi.org/10.1007/s11104-019-04139-1, 2019.
R Core Team: R: A language and environment for statistical computing, R Foundation for Statistical Computing, Vienna, Austria, https://www.R-project.org/ (last access: 10 June 2024), 2021.
Renner, M., Hassler, S. K., Blume, T., Weiler, M., Hildebrandt, A., Guderle, M., Schymanski, S. J., and Kleidon, A.: Dominant controls of transpiration along a hillslope transect inferred from ecohydrological measurements and thermodynamic limits, Hydrol. Earth Syst. Sci., 20, 2063–2083, https://doi.org/10.5194/hess-20-2063-2016, 2016.
Rodriguez, N. B. and Klaus, J.: Catchment Travel Times From Composite StorAge Selection Functions Representing the Superposition of Streamflow Generation Processes, Water Resour. Res., 55, 9292–9314, https://doi.org/10.1029/2019WR024973, 2019.
Salomón, R. L., Peters, R. L., Zweifel, R., Sass-Klaassen, U. G. W., Stegehuis, A. I., Smiljanic, M., Poyatos, R., Babst, F., Cienciala, E., Fonti, P., Lerink, B. J. W., Lindner, M., Martinez-Vilalta, J., Mencuccini, M., Nabuurs, G.-J., van der Maaten, E., von Arx, G., Bär, A., Akhmetzyanov, L., Balanzategui, D., Bellan, M., Bendix, J., Berveiller, D., Blaženec, M., Čada, V., Carraro, V., Cecchini, S., Chan, T., Conedera, M., Delpierre, N., Delzon, S., Ditmarová, L'., Dolezal, J., Dufrêne, E., Edvardsson, J., Ehekircher, S., Forner, A., Frouz, J., Ganthaler, A., Gryc, V., Güney, A., Heinrich, I., Hentschel, R., Janda, P., Ježík, M., Kahle, H.-P., Knüsel, S., Krejza, J., Kuberski, Ł., Kučera, J., Lebourgeois, F., Mikoláš, M., Matula, R., Mayr, S., Oberhuber, W., Obojes, N., Osborne, B., Paljakka, T., Plichta, R., Rabbel, I., Rathgeber, C. B. K., Salmon, Y., Saunders, M., Scharnweber, T., Sitková, Z., Stangler, D. F., Stereńczak, K., Stojanović, M., Střelcová, K., Světlík, J., Svoboda, M., Tobin, B., Trotsiuk, V., Urban, J., Valladares, F., Vavrčík, H., Vejpustková, M., Walthert, L., Wilmking, M., Zin, E., Zou, J., and Steppe, K.: The 2018 European heatwave led to stem dehydration but not to consistent growth reductions in forests, Nat. Commun., 13, 28, https://doi.org/10.1038/s41467-021-27579-9, 2022.
Schoppach, R., Chun, K., He, Q., Fabiani, G., and Klaus, J.: Species-specific control of DBH and landscape characteristics on tree-to-tree variability of sap velocity, Agr. Forest Meteorol., 307, 108 533, https://doi.org/10.1016/j.agrformet.2021.108533, 2021.
Schreel, J. D. M., Steppe, K., Roddy, A. B., and Poca, M.: Does back-flow of leaf water introduce a discrepancy in plant water source tracing through stable isotopes?, Hydrol. Earth Syst. Sci. Discuss. [preprint], https://doi.org/10.5194/hess-2023-13, 2023.
Schwantes, A. M., Parolari, A. J., Swenson, J. J., Johnson, D. M., Domec, J. C., Jackson, R. B., Pelak, N., and Porporato, A.: Accounting for landscape heterogeneity improves spatial predictions of tree vulnerability to drought, New Phytol., 220, 132–146, https://doi.org/10.1111/nph.15274, 2018.
Smith, D. and Allen, S.: Measurement of sap flow in plant stems, J. Exp. Bot., 47, 1833–1844, https://doi.org/10.1093/jxb/47.12.1833, 1996.
Song, L., Zhu, J., Li, X., Wang, K., Wang, G., and Sun, H.: Transpiration of Pinus sylvestris var. mongolica trees at different positions of sand dunes in a semiarid sandy region of Northeast China, Trees-Struct. Funct., 36, 749–762, https://doi.org/10.1007/s00468-021-02247-z, 2022.
Steppe, K., De Pauw, D. J., Doody, T. M., and Teskey, R. O.: A comparison of sap flux density using thermal dissipation, heat pulse velocity and heat field deformation methods, Agr. Forest Meteorol., 150, 1046–1056, https://doi.org/10.1016/j.agrformet.2010.04.004, 2010.
Stojnić, S., Suchocka, M., Benito-Garzón, M., Torres-Ruiz, J. M., Cochard, H., Bolte, A., Cocozza, C., Cvjetković, B., De Luis, M., Martinez-Vilalta, J., Ræbild, A., Tognetti, R., and Delzon, S.: Variation in xylem vulnerability to embolism in European beech from geographically marginal populations, Tree Physiol., 38, 173–185, https://doi.org/10.1093/treephys/tpx128, 2018.
Tai, X., Mackay, D. S., Anderegg, W. R. L., Sperry, J. S., and Brooks, P. D.: Plant hydraulics improves and topography mediates prediction of aspen mortality in southwestern USA, New Phytol., 213, 113–127, https://doi.org/10.1111/nph.14098, 2017.
Thorburn, P. J., Hatton, T. J., and Walker, G. R.: Combining measurements of transpiration and stable isotopes of water to determine ground-water discharge from forests, J. Hydrol., 150, 563–587, https://doi.org/10.1016/0022-1694(93)90126-T, 1993.
Tijdeman, E., Blauhut, V., Stoelzle, M., Menzel, L., and Stahl, K.: Different drought types and the spatial variability in their hazard, impact, and propagation characteristics, Nat. Hazards Earth Syst. Sci., 22, 2099–2116, https://doi.org/10.5194/nhess-22-2099-2022, 2022.
Tromp-van Meerveld, H. J. and McDonnell, J. J.: On the interrelations between topography, soil depth, soil moisture, transpiration rates and species distribution at the hillslope scale, Adv. Water Resour., 29, 293–310, https://doi.org/10.1016/j.advwatres.2005.02.016, 2006.
Tyree, M. and Zimmermann, M. H.: Xylem structure and the ascent of sap, in: 2nd Edn., Springer, https://doi.org/10.1007/978-3-662-04931-0, 2002.
Tyree, M. T. and Yang, S.: Water-storage capacity of Thuja, Tsuga and Acer stems measured by dehydration isotherms – The contribution of capillary water and cavitation, Planta, 182, 420–426, https://doi.org/10.1007/BF02411394, 1990.
Volkmann, T. H., Kühnhammer, K., Herbstritt, B., Gessler, A., and Weiler, M.: A method for in situ monitoring of the isotope composition of tree xylem water using laser spectroscopy, Plant Cell Environ., 39, 2055–2063, https://doi.org/10.1111/pce.12725, 2016.
Walthert, L., Ganthaler, A., Mayr, S., Saurer, M., Waldner, P., Walser, M., Zweifel, R., and von Arx, G.: From the comfort zone to crown dieback: Sequence of physiological stress thresholds in mature European beech trees across progressive drought, Sci. Total Environ., 753, 141 792, https://doi.org/10.1016/j.scitotenv.2020.141792, 2021.
Wassenaar, L., Hendry, M., Chostner, V., and Lis, G.: High Resolution Pore Water δ2H and δ18O Measurements by H2O(liquid)-H2O(vapor) Equilibration Laser Spectroscopy, Environ. Sci. Technol., 42, 9262–9267, https://doi.org/10.1021/es802065s, 2008.
Weemstra, M., Eilmann, B., Sass-Klaassen, U. G., and Sterck, F. J.: Summer droughts limit tree growth across 10 temperate species on a productive forest site, Forest Ecol. Manag., 306, 142–149, https://doi.org/10.1016/j.foreco.2013.06.007, 2013.
Western, A. W., Grayson, R. B., Blöschl, G., Willgoose, G. R., and McMahon, T. A.: Observed spatial organization of soil moisture and its relation to terrain indices, Water Resour. Res., 35, 797–810, https://doi.org/10.1029/1998WR900065, 1999.
Xiao, D., Brantley, S. L., and Li, L.: Vertical Connectivity Regulates Water Transit Time and Chemical Weathering at the Hillslope Scale, Water Resour. Res., 57, 1–21, https://doi.org/10.1029/2020WR029207, 2021.
Zimmermann, J., Link, R. M., Hauck, M., Leuschner, C., and Schuldt, B.: 60-year record of stem xylem anatomy and related hydraulic modification under increased summer drought in ring- and diffuse-porous temperate broad-leaved tree species, Trees, 35, 919–937, https://doi.org/10.1007/s00468-021-02090-2, 2021.